Platinum-gebaseerde katalysatoren op verschillende koolstofdragers en geleidende polymeren voor directe methanol-brandstofceltoepassingen:een overzicht
Abstract
Op platina (Pt) gebaseerde nanodeeltjesmetalen hebben veel aandacht gekregen en zijn de meest populaire katalysatoren voor directe methanolbrandstofcellen (DMFC). De hoge kosten van Pt-katalysatoren, langzame kinetische oxidatie en de vorming van CO-intermediaire moleculen tijdens de methanoloxidatiereactie (MOR) zijn echter grote uitdagingen die samenhangen met enkel-metaal Pt-katalysatoren. Recente studies richten zich op het gebruik van Pt-legeringen, zoals Fe, Ni, Co, Rh, Ru, Co en Sn metalen, of koolstofdragermaterialen om de katalytische prestatie van Pt te verbeteren. In de afgelopen jaren hebben Pt- en Pt-legeringskatalysatoren, ondersteund door een groot potentieel van koolstofmaterialen zoals MWCNT, CNF, CNT, CNC, CMS, CNT, CB en grafeen opmerkelijke interesse gekregen vanwege hun significante eigenschappen die kunnen bijdragen aan de uitstekende MOR en DMFC-prestaties. Dit overzichtsartikel geeft een samenvatting van de ontwikkeling van de bovengenoemde legeringen en ondersteunende materialen die verband houden met het verminderen van het gebruik van Pt, het verbeteren van de stabiliteit en betere elektrokatalytische prestaties van Pt in DMFC. Ten slotte wordt elke katalysator en ondersteuning besproken in termen van morfologie, elektrokatalytische activiteit, structurele kenmerken en de prestaties van de brandstofcel.
Inleiding
Brandstofceltechnologie heeft wereldwijd veel aandacht gekregen. Brandstofcellen (FC's) zijn een veelbelovende alternatieve energieopwekkingstechnologie die chemische energie omzet in elektrische energie door middel van een elektrochemische reactie [1, 2]. Bovendien ligt de focus bij brandstofceltechnologie vooral op het genereren van goedkope productie, waardoor krachtige prestaties van het brandstofcelsysteem worden bereikt en duurzame materialen worden ontdekt. Desalniettemin zijn de veelvoorkomende problemen die zich voordoen in de huidige brandstofceltechnologie dat de systemen hoge intrinsieke kosten en een slechte duurzaamheid met zich meebrengen [1]. Ondanks zijn belofte als brandstofcel, hebben directe methanol-brandstofcellen (DMFC's) uitdagingen en beperkingen, waardoor onderzoekers methoden bestuderen om de DMFC-efficiëntie en prestaties te verbeteren. Veel problemen met DMFC's zijn geïdentificeerd en blijven onopgelost, waaronder cross-over van methanolbrandstof van de anode-elektrode naar de kathode-elektrode [3,4,5] slechte prestaties veroorzaakt door de lage kinetieksnelheid, instabiliteit van de katalysator en thermisch en waterbeheer [6,7,8].
Onlangs zijn er talrijke onderzoeken geweest naar brandstofcellen, waaronder DMFC, protonenuitwisselingsmembraanbrandstofcel (PEMFC), vaste oxidebrandstofcel (SOFC), enzovoort, die populaire brandstofceltechnologieën zijn. Als nieuwe energiebron kunnen DMFC's worden gebruikt voor mobiele en stationaire toepassingen [9, 10]. Er is veel vooruitgang geboekt in het onderzoek op het gebied van brandstofcellen. Onder de brandstofcellen zijn DMFC's de afgelopen jaren uitgebreid bestudeerd [11,12,13,14,15,16] vanwege hun vele voordelen, zoals een hoge vermogensdichtheid, gemakkelijke brandstofverwerking, gemak van opladen en lage milieubelasting. invloed [17, 18]. Verschillende technische uitdagingen voor de commercialisering van DMFC's blijven echter onopgelost, waaronder methanol-crossover, lage chemische reactiesnelheden en katalysatorvergiftiging. DMFC's hebben echter nog steeds de aandacht van veel onderzoekers gekregen en zijn de meest populaire brandstofcellen geworden vanwege hun werking bij lage temperatuur (DMFC-systemen werken bij 373 K). Vanwege de voordelen van DMFC van hoge energie-efficiëntie en een snel opstartsysteem, is DMFC-technologie zeer geschikt om te worden toegepast als stroombronnen voor woningen, batterijen in mobiele apparaten en als brandstof voor voertuigen [19,20,21,22]. Daarnaast zou het concept van DMFC's verder kunnen worden bestudeerd om alternatieve brandstofbronnen zoals aardgas en biomassa te vinden, evenals de vergisting van landbouwproducten om ethanol te produceren, om de afhankelijkheid van onzekere energiebronnen te minimaliseren [14].
In DMFC wordt de anodezijde voorzien van een methanoloplossing die elektro-oxidatie zal ondergaan tot koolstofdioxide (CO2 ) via onderstaande reactie:
$$ {\mathrm{CH}}_3\mathrm{OH}+{\mathrm{H}}_2\to {\mathrm{CO}}_2+6{\mathrm{H}}^{+}+6{ \mathrm{e}}^{\hbox{-} } $$ (1)Terwijl aan de kathodezijde het proton, de zuurstof (uit lucht) wordt gereduceerd tot water:
$$ 3/2\ {\mathrm{O}}_2+6{\mathrm{H}}^{+}+6\ {\mathrm{e}}^{\hbox{-}}\to 3{\ wiskunde{H}}_2\mathrm{O} $$ (2)De nettovergelijking DMFC-reactie kan als volgt worden samengevat:
$$ {\mathrm{CH}}_3\mathrm{OH}+3/2{\mathrm{O}}_2\to {\mathrm{CO}}_2+2{\mathrm{H}}_2\mathrm{ O} $$ (3)In DMFC-systemen zijn er twee soorten DMFC-modi:actieve en passieve modi [23,24,25]. In een actief DMFC-systeem wordt de uitlaatstroom van de DMFC-stack gerecirculeerd door de gesloten-lusregeling van de vloeibare methanoltoevoer. Ondertussen wordt de vloeibare methanol bij de anodestroom geregeld door een methanolconcentratiesensor die een belangrijke rol speelt bij het leveren van voldoende injectie van extra methanol en water om deze brandstof te herstellen op basis van de doelconcentratie. Er zijn verschillende soorten methanolconcentratiesensoren die in het DMFC-systeem worden gebruikt om de methanoltoevoerconcentratie te regelen en te handhaven [17]. Gewoonlijk wordt de vloeibare methanol aan de anodezijde geleverd door een peristaltische pomp, terwijl de omringende lucht die zuurstof bevat door een blazer of ventilator aan de kathodezijde wordt toegevoerd [16]. In een passieve modus van het DMFC-systeem wordt de vloeibare methanol continu aan het systeem toegevoerd. Dit passieve concept is zeer aantrekkelijk voor het DMFC-systeem [26,27,28]. Het concept van passief betekent dat het systeem volledig autonoom werkt zonder enige ondersteunende apparaten. Het concept van passieve DMFC betekent dat het systeem volledig autonoom werkt zonder enige hulp van een extern apparaat om methanol te pompen en lucht in de stack te blazen. In de passieve modus van het DMFC-systeem wordt de katalysatorlaag geleverd door methanol en zuurstof als reactanten. Tijdens de methanoloxidatiereactie (MOR), CO2 en water zal passief uit de cel worden verwijderd, d.w.z. diffusie, natuurlijke convectie, capillaire werking, enz. [20]. Passieve modus DMFC lijkt voordeliger in vergelijking met de actieve modus DMFC in termen van eenvoudiger, compacter ontwerp en lage kosten. Het complexe systeemontwerp en de besturing kunnen een nadeel zijn van actieve modus DMFC [21]. Vanuit het oogpunt van praktisch gebruik lijkt DMFC met actieve modus geschikter te zijn in systemen met hoog vermogen, terwijl DMFC in passieve modus meer geschikt is om te worden gebruikt in vereisten met laag vermogen [22].
Figuur 1 toont de opzet en het ontwerp voor een eencellige DMFC. Een eencellige DMFC-stack bestaat uit een vijflaags membraanelektrodesamenstel (MEA) dat is ingeklemd tussen twee platen die anode en kathode zijn. Aan de anodezijde wordt vloeibare methanol (bevat methanol en gedeïoniseerd water) en absolute methanol door een peristaltische pomp in het kanaal gestroomd. Aan de kathodezijde wordt door een rotameter lucht in de brandstofcel gepompt. De temperatuurregelaar in de DMFC-stack wordt gebruikt om de werktemperatuur in de cel te handhaven door een aanvullend verwarmingsapparaat. Het elektronische laadapparaat wordt gebruikt om de stroomdichtheid in verschillende niveaus te veranderen en de overeenkomstige waarden van de spanning te meten. De celprestaties worden gecontroleerd door een elektrochemisch werkstation, terwijl de productie van CO2 als eindproduct van de totale reactie wordt gemeten met een CO2 concentratiedetector [23]. In directe methanol-brandstofcellen zijn er verschillende belangrijke bedrijfsparameters waarmee rekening moet worden gehouden tijdens experimenteel onderzoek, namelijk (i) werktemperatuur, (ii) methanolconcentratie en (iii) invoerstroomsnelheden van de methanoloplossing en lucht [23] . Afbeelding 2a, b toont respectievelijk de actieve en passieve modus van de DMFC.
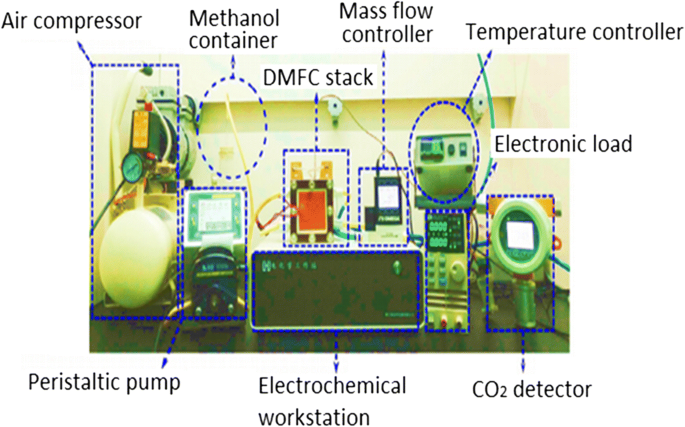
Een algemene experimentele opstelling voor single cell DMFC [23]
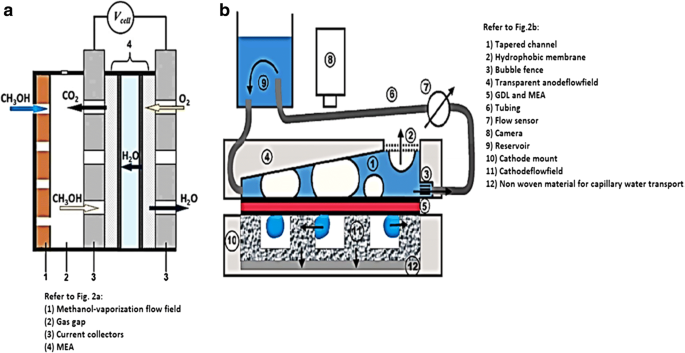
Schematisch diagram van a actieve modus [24] en b passieve modus [25] van DMFC
Deze beoordeling zal zich richten op de recente vooruitgang in de onderzoeken en ontwikkelingen van katalysatordrager op basis van Pt-katalysator als de edele katalysator in DMFC. We omvatten de activiteit van op Pt gebaseerde katalysatoren gecombineerd met legeringen, metalen, overgangsmetalen, metaalcarbiden, metaalnitriden en verschillende koolstofhoudende soorten, zoals grafeen/grafeenoxide (G/GO), koolstof nanobuis (CNT), koolstof nanovezel ( CNF), carbon nanocoil (CNC), carbon black (CB), multiwall carbon nanotube (MWCNT) en carbon mesoporous (CMS) dragers, evenals geleidende polymeren, zoals polyaniline (PANi) en polypyrrool (Ppy) als drager materiaal. Er kunnen veel synthesemethoden worden toegepast om op Pt gebaseerde katalysatoren te bereiden. De meest gebruikte methoden om Pt-deeltjes op nanoschaal te verkrijgen zijn impregnatie [29,30,31,32,33,34], hydrothermische technieken [35,36,37,38,39,40,41], micro-emulsie [42,43, 44,45] en reductie [46, 47]. In het algemeen kan de bereidingsmethode de morfologie en grootte van de katalysatordeeltjes beïnvloeden; daarom is de selectie van de methode voor katalysatorsynthese erg belangrijk.
Prestaties van verschillende soorten Pt-gebaseerde katalysatoren
In het afgelopen decennium hebben veel onderzoekers hun onderzoek gericht op de ontwikkeling van elektrokatalysatoren om de elektrokatalytische activiteit ervan in methanol-MOR voor DMFC-systeem te verbeteren [37, 38]. Platina (Pt) is een enkelmetaalkatalysator die een significant hoge katalytische activiteit voor de MOR vertoont. Pt alleen in een DMFC-systeem kan echter gemakkelijk worden vergiftigd door de intermediaire soorten, dwz koolmonoxide (CO), en de hoge kosten van de Pt-katalysator beperken de commerciële toepassing ervan als elektrokatalysator, waardoor de kinetische snelheid van methanoloxidatie wordt verlaagd. in DMFC-systeem [48,49,50]. Deze drie punten zijn de belangrijkste obstakels en beperkingen van het gebruik van Pt alleen als elektrokatalysator voor DMFC. Om deze hindernissen te overwinnen, zijn er echter verschillende onderzoeken uitgevoerd om op Pt gebaseerde elektrokatalysatoren te synthetiseren om betere elektrokatalytische prestaties te bereiken met minder Pt-gebruik [11, 47, 51, 52]. Normaal gesproken kan de gemiddelde grootte van Pt-deeltjes en de morfologie ervan worden bepaald door middel van scanning-emissiemicrofoto (SEM) of transmissie-elektronenmicrofoto (TEM) -analyse, de meest gebruikelijke methoden in het katalyseveld die kunnen worden gebruikt om de fysieke eigenschappen van elektrokatalysatoren te karakteriseren. Tabel 1 toont de gemiddelde deeltjesgrootte van de Pt-deeltjes met verschillende synthesemethoden, eigenschappen en hun prestaties.
Bimetaal PtRu wordt beschouwd als de meest actieve katalysator vanwege het bifunctionele mechanisme en de ligandeffecten [48, 53]. PtRu wordt een interessante katalysatorlegering en wordt tot op heden gebruikt met veel koolstofdragers. Het toxicologische effect van de toevoeging van ruthenium (Ru) metaal blijft echter onzeker [49]. Daarom is er onderzoek gedaan naar goedkopere legeringen die Pt mengen met andere niet-edele metalen [49,50,51,52, 54,55,56,57], zoals besproken in de sectie "Prestaties van op Pt gebaseerde legeringen".
Prestaties van op Pt gebaseerde legeringen
Arico et al. [35] ontdekte dat er talloze onderzoeken zijn uitgevoerd om de katalytische activiteit van Pt-katalysatoren in de MOR te verhogen. In veel onderzoeken is de optimale Pt-Ru-verhouding geïdentificeerd als 1:1, en deeltjesgroottes op nanoschaal zijn de ideale grootte om het gebruik van de katalysator te verbeteren. Echter, Shi et al. [38] identificeerden dat 3:2 de optimale verhouding was voor Pt-Ru in hun experimenten om de katalytische activiteit van MOR te verbeteren. Afgezien daarvan kan de elektrokatalytische activiteit voor de elektro-oxidatie-activiteit van methanol ook worden verhoogd als de PtRu-elektrokatalysatordeeltjes nanodeeltjes zijn in het bereik van 2-4 nm. Paulas et al. [39] was het met deze stelling eens. Zoals we weten, vertoont Pt een hoge reactiviteit ten opzichte van methanolbrandstof, waardoor Pt-metaal een ideale elektrokatalysator is voor de anode-elektrode in het DMFC-systeem. Niettemin zal tijdens MOR van de Pt-katalysator zich koolmonoxide (CO), d.w.z. de intermediaire soort, vormen op het oppervlak van Pt-deeltjes, wat dus het katalysatoroppervlak vergiftigt [58,59,60,61]. Er zijn dus enige inspanningen nodig om het probleem te overwinnen dat verband houdt met de vorming van giftige soorten op het oppervlak van de Pt-deeltjes, zodat ze de actieve plaatsgebieden van Pt niet bedekken. Over het algemeen zijn binaire legeringen, zoals PtRu [62,63,64,65,66], PtRh [67,68,69,70,71], PtAu [72,73,74], PtSn [62, 63, 75, 76,77], PtNi [64,65,66,67,78,69], PtCo [70, 71, 78,79,80] en PtFe [81,82,83,84,85] worden vaak gebruikt als elektrokatalysatorcombinaties voor de anode-elektrode in het DMFC-systeem. Aangenomen wordt dat de toevoegingen van deze metalen, zoals ruthenium (Ru), tin (Sn) en rhodium (Rh), een hogere katalytische activiteit opleveren.
Opname van nikkel (Ni) in op platina gebaseerde katalysator geeft superieure prestaties voor MOR en DMFC. In het laatste onderzoek leggen Guerrero-Ortega en collega's uit dat de toevoeging van Ni in de Pt-Vulcan-ondersteuning een belangrijke toename in de faradische stroom tijdens MOR van één orde van grootte bevordert, hoewel het gebruik van Pt lager is in de bimetaalkatalysator [ 55] . Hun experimentele resultaten suggereerden ook dat de toevoeging van Ni enkele structurele en elektronische modificaties bevordert die een betere reactieprestatie aan de elektrode-interface verbeteren. In een ander werk verbeterde de opname van Au in een Pt-legering de elektrokatalytische activiteiten vanwege de veranderende elektronische structuur en de verbetering van het elektrochemisch actieve gebied (ECSA) [47]. Terwijl de toevoeging van tin (Sn) aan een op Pt gebaseerde legering een toename in elektrokatalytische activiteit liet zien, die sterk wordt beïnvloed door de opname van Sn in het legeringssysteem en geoxideerde vormen, waardoor de reactie gemakkelijker wordt gestimuleerd vanwege het lagere oxidatiepotentieel [ 56]. Ook verbeterde de toevoeging van kobalt (Co) aan een op Pt gebaseerde legering de katalytische eigenschappen aanzienlijk door PtCo (1:9)/rGO-katalysator die tien keer hoger bleek te zijn dan Pt/rGO [51]. De toename in stroomdichtheid wordt toegeschreven aan een hogere dispersie van PtCo-nanodeeltjes op de hydrofiele aard van rGO-ondersteuning die wateractivering bevordert en leidt tot het oxideren van de COadvertenties op Pt-sites. Bovendien bevordert het volgens het bifunctionele mechanisme van Co de H2 O activering waardoor meer -OH ionen en andere O2 . ontstaan -bevattende soorten om CO-tussenproducten op de Pt-site te oxideren [57]. Dit bifunctionele mechanisme van Co zou ook kunnen worden gebruikt voor andere katalytische overgangsmetalen naar MOR. Het katalytische oxidatiemechanisme voor CO-soorten tot CO2 in aanwezigheid van PtCo-katalysatoren kan als volgt worden samengevat:
$$ \mathrm{Pt}+{\mathrm{CH}}_3\mathrm{OH}\to \mathrm{Pt}\hbox{-} {\mathrm{CO}}_{\mathrm{ads}}+4 {\mathrm{H}}^{+}+4{\mathrm{e}}^{\hbox{-} } $$ (4) $$ \mathrm{Co}+{\mathrm{H}}_2\ mathrm{O}\to \mathrm{Co}{\left(\mathrm{OH}\right)}_{\mathrm{ads}}+{\mathrm{H}}^{+}+{\mathrm{e }}^{\hbox{-} } $$ (5) $$ {\mathrm{PtCO}}_{\mathrm{ads}}+\mathrm{Co}{\left(\mathrm{OH}\right) }_{\mathrm{ads}}/{\mathrm{CO}}_2+\mathrm{Pt}+\mathrm{Co}+{\mathrm{H}}^{+}+{\mathrm{e}}^ {\hbox{-} } $$ (6)Verder hebben Löffler et al. [86] heeft met succes PtRu gesynthetiseerd als de anodekatalysator voor DMFC's waarmee de meest actieve elektrokatalysator voor methanolelektro-oxidatie werd geproduceerd bij ongeveer 50 at.% Ru. Ondertussen hebben Dinh et al. rapporteerde [87] dat PtRu met een verhouding van PtRu 1:1 een sterker metallisch gedrag en een hogere elektrokatalytische activiteit voor methanoloxidatie (MOR) heeft. De prestaties zijn gerelateerd aan deze twee belangrijke factoren:(i) gemaximaliseerd katalysatoroppervlak en (ii) katalysatoroppervlak met een maximaal aantal metaallegeringsplaatsen met een atomaire verhouding dichtbij 1:1. Beide groepen lieten ook een hoge score zien. Op basis van het bifunctionele mechanisme, Aricò et al. [58] en Goodenough et al. [62] suggereerde dat de CO-tussenvormen die zich op de oppervlakte-actieve plaatsen van Pt hebben gevormd, kunnen worden geoxideerd tot koolstofdioxide (CO2 ) door actieve zuurstofatomen gevormd op de secundaire elementen, bijvoorbeeld Ru, Sn en Mo, in het lagere potentiaalgebied. Tabel 1 vatte de prestaties samen van verschillende soorten Pt-legeringskatalysatoren, uitgevoerd door onderzoekers voor MOR. Volgens het bifunctionele mechanisme [88,89,90] kan de MOR op gedragen PtRu-legeringskatalysatoren worden samengevat als de volgende vergelijking. Pt is een actievere katalysator voor de methanoladsorptie dan Ru. Daarom gehoorzaamt de algehele reactie op PtRu-elektrokatalysatoren voor de oxidatiereactie van methanol aan het bifunctionele mechanisme.
$$ \mathrm{Pt}+{\mathrm{CH}}_3\mathrm{OH}\to \mathrm{Pt}\hbox{-} {\mathrm{CH}}_3\mathrm{OH}\mathrm{ads }\naar \mathrm{Pt}\hbox{-} {\mathrm{CO}\mathrm{H}}_{\mathrm{ads}}\naar 3\mathrm{H}+3\mathrm{e}\hbox {-} \naar \mathrm{Pt}\hbox{-} {\mathrm{CO}}_{\mathrm{ads}}+{\mathrm{H}}^{+}+{\mathrm{e}} ^{\hbox{-} } $$ (7) $$ \mathrm{Ru}+{\mathrm{H}}_2\mathrm{O}\to \mathrm{Ru}\hbox{-} {\mathrm{ OH}}_{\mathrm{ads}}+{\mathrm{H}}^{+}+{\mathrm{e}}^{\hbox{-} } $$ (8) $$ \mathrm{Pt }\hbox{-} {\mathrm{CO}\mathrm{H}}_{\mathrm{ads}}+\mathrm{Ru}\hbox{-} {\mathrm{OH}}_{\mathrm{ads }}\naar \mathrm{Pt}+\mathrm{Ru}+{\mathrm{CO}}_{2+}2{\mathrm{H}}^{+}+2{\mathrm{e}}^ {\hbox{-} } $$ (9) $$ \mathrm{Pt}\hbox{-} {\mathrm{CO}}_{\mathrm{ads}}+\mathrm{Ru}\hbox{-} {\mathrm{OH}}_{\mathrm{ads}}\to \mathrm{Pt}+\mathrm{Ru}+{\mathrm{CO}}_2+{\mathrm{H}}^{+}+{ \mathrm{e}}^{\hbox{-} } $$ (10)Verwijzend naar dit bifunctionele mechanisme, wordt methanol aanvankelijk gedissocieerd en geadsorbeerd op Pt, vervolgens ontleed tot COads en/of formyl-achtige soorten -CHOadvertenties door dehydrogeneringsreactie (7). Tegelijkertijd dissocieert water tot OHadvertenties en geadsorbeerd op Ru-plaatsen (8). Vervolgens adsorbeerde de soort op Pt- en Ru-sites en combineerde ze samen om CO2 . te vormen molecuul (9) en (10). De reactie tussen Pt–COadvertenties en Ru–OHads leidt tot CO2 evolutie, het genereren van vernieuwde Pt- en Ru-sites (reactie 10). Terwijl een ander werk van Ewelina Urbanczyk et al. [48] voerde de methanoloxidatiereactie uit voor PtNi-katalysator in alkalisch medium (1,0 M KOH). Theoretisch is de methanoloxidatiereactie in alkalisch milieu:
$$ {\mathrm{CH}}_3\mathrm{OH}+6\mathrm{OH}\to {\mathrm{CO}}_2+5{\mathrm{H}}_2\mathrm{O}+6{ \mathrm{e}}^{\hbox{-} } $$De reactie begint bij de Pt-elektrode van de DMFC tot koolstofdioxide. Tijdens dit proces kunnen intermediaire moleculen (CO) worden gevormd die gif en deactivering van de Pt-actieve kant kunnen veroorzaken. Dit CO-molecuul is een product van onvolledige oxidatie van methanol. De onvolledige oxidatie van methanol vormt het CO als tussenproduct (vergelijking 11). Het oppervlak van de elektrokatalysator kan ook hydroxylgroepen adsorberen (Vgl. 6). Ten slotte wordt door de desorptie van het hoofdproduct koolstofdioxide gevormd (13). Het tweede gif dat tijdens de methanoloxidatie kan ontstaan, is methaan. In dit geval kan de volgende reactie optreden (8). De totale oxidatie van de tussenvorm van koolstof tot koolstofdioxide in de elektrochemische reactie is als volgt:
$$ 3\mathrm{Pt}+{\mathrm{CH}}_3\mathrm{OH}\to \mathrm{Pt}-\mathrm{COads}+4{H}^{+}+2\mathrm{Pt }+4e-+{H}^2O $$ (11) $$ \mathrm{Ni}+{H}_2O\to \mathrm{Ni}-\mathrm{OHads}+{H}^{+}+e - $$ (12) $$ \mathrm{Pt}-{\mathrm{CO}}_{\mathrm{ads}}+\mathrm{Ni}-\mathrm{OHads}\to {\mathrm{CO}} _2+{H}^{+}+\mathrm{Pt}+\mathrm{Ni}+e- $$ (13) $$ \mathrm{Pt}-{\mathrm{CH}}_3+\mathrm{Pt}- H\to 2\ \mathrm{Pt}+{\mathrm{CH}}_4 $$ (14) $$ \mathrm{Pt}-{\mathrm{CH}}_3+\mathrm{Ni}{\left(\ mathrm{OH}\right)}_2\to \mathrm{Pt}+{\mathrm{CO}}_2+\mathrm{Ni}+5{H}^{+}+5e- $$ (15)Momenteel bestuderen onderzoekers nog steeds legeringstechnieken om de katalytische activiteit van op Pt gebaseerde elektrokatalysatoren te verbeteren door ternaire en quaternaire legeringen van Pt te fabriceren, zoals PtRuSn [91, 92], PtRuNi [93,94,95], PtRuMo [70, 96 , 97], quaternaire PtRuOsIr [79, 80] en PtRuIrSn [97, 98], vanwege hun uitstekende gedrag in MOR en verwijdering van de intermediaire soorten (CO) die zich vormen op de Pt-oppervlakte. Maar de toevoeging van het derde en vierde metaal in deze ternaire en quaternaire katalysatoren is nog onbekend. Bovendien zijn er enkele beperkingen en uitdagingen bij het produceren van de ternaire en quaternaire legeringen. De optimalisatie van katalysatormorfologie en katalysatorsamenstellingen wordt moeilijk te verkrijgen vanwege de vele mogelijke combinaties van metalen en samenstellingen. Veel onderzoeken hebben echter aangetoond dat de toevoeging van derde en vierde metaal de katalytische activiteit opmerkelijk verhoogde, de stabiliteit van de katalysator verhoogde en een goede CO-tolerantie voor methanolelektro-oxidatie en DMFC-toepassingen.
Tsiouvaras et al. [99] voerde de elektrochemische meting van PtRuMo/C-katalysatoren uit en ontdekte dat hoewel alle ternaire katalysatoren actiever waren in de richting van CO- en methanoloxidatie dan de binaire katalysator, de met H2 behandelde katalysator vertoonde een verbeterde prestatie met ongeveer 15% met betrekking tot de ternaire katalysatoren die in He of zonder behandeling waren behandeld. In 2012 Hu et al. [100] heeft met succes een superieure bimetaal (PtNi) elektrokatalysator gesynthetiseerd, namelijk holle mesoporeuze PtNi-nanosferen (HMPNN's). De katalysator vertoonde uitstekende katalytische prestaties in de MOR met aanzienlijk verbeterde Pt-gebruiksefficiëntie vanwege de unieke structuur van HMPNN's en hun grote elektrochemische oppervlak. Terwijl rond 2016 het werk van Yang et al. [101] onderzochten ook de reactiviteit van gesynthetiseerde bimetalen PtFe-elektrokatalysatoren waarin ze zagen dat de sterke interactie tussen Pt en ijzer (Fe) metalen de adsorptie-energieën van bimetalen NP's kan verminderen. Ze ontdekten ook dat de bimetalen PtFe-nanodeeltjes er de voorkeur aan geven te worden geadsorbeerd op het enkele lege grafeen via de Fe-atomen wanneer Pt- en Fe-atomen zich beide op het oppervlak bevinden, omdat de interacties tussen Fe-atomen en grafeen met één vacature sterker zijn dan die tussen Pt-atomen en enkele vacature grafeen. Figuur 3 illustreert de positie van Pt- en Fe-deeltjes gedispergeerd op de grafeendrager zoals gesuggereerd door Yang et al. [101].
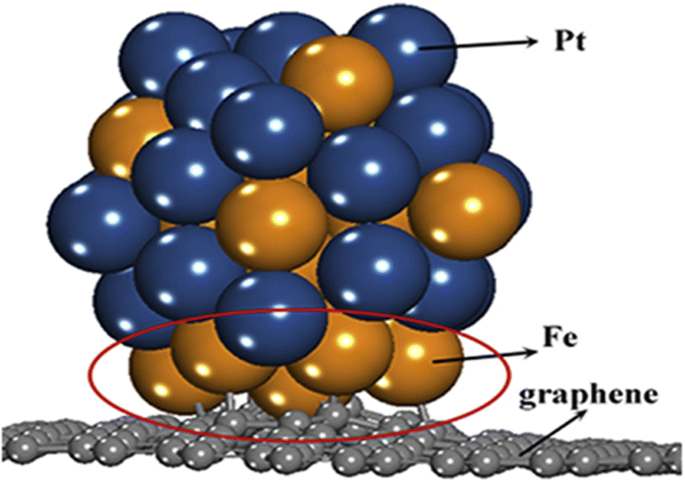
De positie van PtFe-katalysator op grafeendrager geïllustreerd door Yang et al. [101]
Prestaties van op Pt gebaseerde katalysator en overgangsmetaalcarbide
Overgangsmetaalcarbide (TMC), met hoge mechanische en chemische stabiliteit tegen corrosie, goede weerstand tegen zure omgevingen, stabiliteit op lange termijn en hoge CO-tolerantie, kan fungeren als anodekatalysatoren [88,89,90, 102,103,104]. Daarnaast bieden TMC's ook veel voordelen ten opzichte van hun moedermetalen met betrekking tot de activiteit, selectiviteit en weerstand tegen gif, bijvoorbeeld wolfraamcarbide (WC) vertoont speciale eigenschappen, zoals goede elektrische geleidbaarheid, weerstand tegen zure omgevingen, lage kosten , en tolerantie voor CO-vergiftiging in het elektro-oxidatieproces van methanol [88, 105, 106].
Wang et al. [103] rapporteerde de synthese van een groot oppervlak (256 m 2 g −1 ) wolfraamcarbide microsferen via een eenvoudige hydrothermische methode. W2 C werd gevonden als de hoofdfase in het gesynthetiseerde monster. Momenteel onderzoeken onderzoekers momenteel het potentieel van Pt ondersteund op WC als een ideale katalysator voor DMFC [38, 88, 107, 108]. Christiaan et al. [106] concludeerde dat TMC's zich ten opzichte van hun overgangsmetaalelementen gedragen als edele metalen zoals Pt, Pd, Rh en Ru voor bepaalde chemische en elektrochemische reacties, waaronder oxidatiereactie van waterstof, koolmonoxide en alcohol en reductie van zuurstof [109, 110]. In een andere studie, Liu et al. [107] presenteerde dat molybdeencarbiden (Mo-Carbides) zouden kunnen werken als promotors voor wolfraamcarbide en de elektrokatalytische activiteit in de DMFC kunnen verhogen. Zonder de opname van Pt-metaal is de elektrokatalytische activiteit van zuiver WC naar de MOR voor een DMFC-systeem echter nog steeds laag. Daarom is een kleine hoeveelheid platinametaal toegevoegd aan de WC-component erg handig om het voordeel van het synergetische effect tussen Pt en WC te verkrijgen [91, 111, 112]. Ondertussen hebben Hassan et al. [109] onthulde dat de gewone onzuiverheid (CO-soort) die zich vormt tijdens de oxidatie van methanol een sterke bindingsenergie heeft op het Pt-oppervlak; daarom moet het worden geoxideerd zodat het van de Pt-actieve plaatsen kan worden verwijderd. De toevoeging van WC-component in Pt/WC-elektrokatalysator heeft een hoge CO-tolerantie voor MOR aangetoond, wat wijst op het bestaan van synergetische effecten tussen het Pt-metaal en WC als ondersteunende component. De studie werd ook uitgevoerd door een andere onderzoeker door minder Pt-metaal te gebruiken om de kosten van Pt te verlagen, terwijl de elektrokatalytische prestatie goed bleef.
Verder is de WC-component actiever voor de vorming van een methoxygroep (CH3 O-) dan zuiver Pt [113, 114]. CV-prestaties van (Pt:Ru)4-WC/RGO tonen uitstekende katalytische prestaties met een stroomdichtheid van 330,11 mA mg −1 Pt vergeleken met de andere vijf katalysatoren, wat aangeeft dat de als gesynthetiseerde elektrokatalysator een uitstekende katalytische activiteit heeft ten opzichte van de MOR. Bovendien verhoogde de combinatie van Ru en WC op de Pt-katalysator de hoeveelheid OH-oppervlakte en maakte het mogelijk dat de op het Pt-oppervlak geadsorbeerde CO bij lagere potentialen werd geoxideerd [39].
Prestaties van op Pt gebaseerde katalysator en overgangsmetaalnitride
Overgangsmetaalnitride (TMN) is een ideale kandidaat als Pt-katalysatordrager vanwege zijn goede elektrische geleidbaarheid (metaal), hardheid, hoge elektrochemische stabiliteit en corrosieweerstand onder bedrijfsomstandigheden met brandstofcellen [115,116,117,118]. Overgangsmetaalnitriden, zoals CrsN, TiN en VN, gedragen Pt-katalysatoren zijn gerapporteerd en vertoonden hoge katalytische prestaties en betere stabiliteit in vergelijking met de traditionele koolstofdragers [112]. Alle overgangsmetalen kunnen nitride vormen, behalve de tweede en derde rij van metalen uit groep 8, 9 en 10 (Ru, Os, Rh, Ir, Pd en Pt). Het gedrag en de structurele eigenschappen van overgangsmetaalnitriden zijn te vinden in de literatuur [92,93,94]. Xiao et al. [112] bereidde een door titanium-kobaltnitride ondersteunde Pt-elektrokatalysator die uitstekende prestaties en stabiliteit vertoonde ten opzichte van de zuurstofreductiereactie (ORR). De Ti0.9 Co0.1 Pt-katalysator op N-drager vertoonde een kleine deeltjesgrootte en een goede metaaldispersie. Deze geprepareerde elektrokatalysator handhaafde ook het elektrochemische oppervlak (ECSA) van Pt en verbeterde de ECSA-conservering aanzienlijk, met slechts een afname van 35% in de vroege ECSA-daling na 10.000 ADT-cycli. Kobaltdoping verbeterde de ORR-activiteit en duurzaamheid aanzienlijk. Ondertussen kan een hoogwaardige en duurzame elektrokatalysator in het DMFC-systeem worden verkregen met behulp van een Pt(Ru)/TiN-elektrokatalysator met een groot oppervlak, die ook een hoge elektrochemische activiteit ten opzichte van MOR vertoonde met een verbetering van ∼ 52% van de katalytische activiteit en een goede stabiliteit/duurzaamheid vergeleken met naar commerciële JM-Pt(Ru). Ondertussen bereikten de eencellige prestaties van de DMFC een betere maximale vermogensdichtheid met 56% en vertoonden ze een uitstekende elektrochemische stabiliteit voor CSG-Pt(Ru)/TiN-elektrokatalysator [115].
Lopend onderzoek naar Pt-nanodeeltjes ondersteund op titanium-ijzernitride-nanobuizen met holle en poreuze structuur en een groot oppervlak werd gesynthetiseerd door Li et al. [116]. Het vertoonde een significante toename van de elektrokatalytische activiteit ten opzichte van de MOR in zure toestand en had een betere duurzaamheid. De redenen voor deze eigenschappen waren te wijten aan hun experimentele gegevenswerk dat verifieerde dat de toevoeging van Fe de elektronische structuur van Pt-atomen kan afstemmen, wat bijdraagt aan de versterkte activiteit en stabiliteit van de Pt-katalysator voor de MOR. Ondertussen, in eerder werk van Xiao et al. [117], Pt/Ti0,8 Ma0,2 N-katalysator vertoonde een poreuze structuur en een groot oppervlak, kleine en goed gedispergeerde Pt-nanodeeltjes. Dit katalysatorsysteem behield de intrinsieke elektrochemische stabiliteit van de TiN-nanostructuur en verbeterde opmerkelijk de MOR-activiteit en duurzaamheid. De momenteel beschikbare informatie over de elektrochemische stabiliteit van wolfraamnitride (WN) is echter nog steeds onvoldoende [109].
Ondertussen, MoxN (x = 1 of 2) op Ti-substraat vertoonde elektrochemische stabiliteit in een zure elektrolyt van 4,4 M H2 SO4 tot anodepotentiaal van +-0,67 V (vs. SHE) over 50 herhaalde cycli [110]. Deze elektrokatalysator vertoonde echter oppervlakteschade, zoals barsten en afbrokkelen, in de hoog kathodische (onder -0,1 V vs. SHE) en anodische (boven + -0,67 V vs. SHE) potentiële gebieden als gevolg van respectievelijk kathodische en anodische corrosie. In het hoge anodepotentiaalgebied boven +-0,67 V (vs. SHE), nam de zuurstofsamenstelling toe als gevolg van MoOx-oxidevorming, wat deactivering zou kunnen veroorzaken. Deze resultaten geven aan dat MoxN reageert met de zuurstofspecies die aanwezig zijn in de waterige elektrolyt en onstabiel is boven +-0,67 V (vs. SHE). Mustafha et al. [111] ontdekte dat Pt geladen op TiN als drager elektroactiviteit vertoonde voor de methanoloxidatie, met een hoge If/Ib-verhouding die een hoge CO-weerstand vertegenwoordigt in het voltammogram uitgevoerd met een scansnelheid van 20 mV/s in 0,5 M CH 3 OH + 0,5 M H2 SO4 als de elektrolyt. Als oorzaak van de CO-resistentie van Pt/TiN werd het bifunctionele effect tussen Pt en TiN genoemd. Bovendien, Ottakam Thotiyl et al. [91] behaalde goede resultaten voor een met Pt beladen TiN-katalysator, met een zeer goede CO-tolerantie voor de elektrochemische oxidatie van methanol. Ze concludeerden dat de speciale eigenschappen van TiN die het geschikt maakten als een Pt-drager voor de MOR in een alkalisch medium, zijn dat het uitzonderlijke stabiliteit, extreme corrosieweerstand, goede elektronische geleidbaarheid en sterk hechtgedrag vertoont. TiN-ondersteunde katalysatoren zijn gunstig in termen van stabiliteit op lange termijn, uitwisselingsstroomdichtheid en stabiele stromen bij lage overpotentiaal. In de experimenten werden platinaladingen van 40 gew.% op TiN gebruikt.
In recent years, Liu et al. [118] successfully synthesized platinum on titanium nickel nitride decorated 3D carbon nanotubes which reduced graphene oxide (TiNiN/CNT-rGO) support by solvothermal process followed by nitriding process. Pt with small particle size is well-dispersed on TiNiN/CNT-rGO support. The 3D shape of CNT-rGO support gives a fast route for charge transfer and mass transfer as well as TiNiN NPs with good synergistic effect and the strong electronic coupling between different domains in TiNiN/CNT-rGO support. Thus, it greatly improved the catalytic activity of this catalyst. In another research, the non-carbon TiN nanotubes-supported Pt catalyst done by Xiao et al. [119] also displayed enhanced catalytic activity and durability toward MOR compared with the commercial Pt/C (E-TEK) catalyst.
Performance of Pt-Based Catalysts with Transition Metal Oxide
Pan et al. [92] reported the synthesis of platinum–antimony-doped tin oxide nanoparticles supported on carbon black (CB) as anode catalysts in DMFC, which exhibited better improvement in catalytic activity toward MOR compared to Pt-SnO2 /C or commercial Pt/C electrocatalyst. The enhancement in activity was attributed to the high electrical conductivity of Sb-doped SnO2 , which induced electronic effects with the Pt catalysts. Another work done by Abida et al. [93]described the preparation of Pt/TiO2 nanotube catalysts for methanol electrooxidation. The TiO2 nanotubes-supported Pt catalyst (Pt/TiO2 nanotubes) exhibited excellent catalytic activity toward MOR and had good CO tolerance. They also reported that the use of hydrogenotitanate nanotubes as a substrate for the Pt catalyst considerably improved the COads oxidation on Pt, but the MOR still occurred at high potential. Then, several years later, Wu et al. [94] synthesized Pt-C/TiO2 with MOR activity 1.6 higher than commercial Pt-C and the stability of Pt-C/TiO2 was also enhanced by 6.7 times compared to Pt-C. The excellent performance of this catalyst was a contribution of mesopores and partially coated carbon support. Zhou et al. [95] prepared hollow mesoporous tungsten trioxide microspheres (HMTTS) using the spray-drying method to yield Pt/HMTTS. The electrocatalyst exhibited excellent electrocatalytic activity and high stability toward MOR than Pt/C and Pt/WO3 electrocatalysts, which may be attributed to the well-ordered Pt particles (with an average size of 5 nm) on the HMTTS surface. Wu et al. [120] used polystyrene spheres as templates to obtain pore-arrayed WO3 (p-WO3 ). The Pt nanoparticles with an approximate size of 3.3 nm dispersed on pore-arrayed WO3 (Pt/p-WO3 ) exhibited high catalytic activity toward MOR.
Li et al. [121] used Sn-doped TiO2 -modified carbon-supported Pt (Pt/Ti0.9 Sn0.1 O2 –C) as an electrocatalyst for a DMFC system. The synthesized Pt/Ti0.9 Sn0.1 O2 –C electrocatalyst revealed high catalytic activity and CO tolerance toward MOR. The enhanced catalyst activity was due to the high content of OH groups on the Ti0.9 Sn0.1 O2 electrocatalyst sample and the strengthened metals and support interactions. In addition, Lv et al. [122] also reported in their work that the addition of TiO2 could not only facilitate CO removal and hinder CO formation on Pt surface during methanol oxidation, but it can also prevent the agglomeration and corrosion of Pt, which can be concluded from strong metal-supports interaction between TiO2 –C and Pt. Huang et al. [123] revealed that a TiO2 -coated carbon nanotube support for Pt electrocatalysts could be prepared via a one-step synthesis. Hao et al. [124] developed a new catalyst composed of Pt nanoparticles deposited on graphene with MoO3 . These catalysts exhibited high catalytic activity toward MOR and high resistance to CO species. However, the size of MoO3 must be tuned by controlling the metal oxide loading.
The selection of metal oxide such as MnO, RuO, CeO, SnO2 , MgO, and V2 O5 as additional component in electrocatalyst of Pt because of their low cost, good electrochemical properties, and have proton-electron intercalation properties [125]. From the catalytic activity aspect, it can be summarized that the addition of these metal oxides can enhance the electrocatalytic activity of DMFC and other fuel cells. The incorporation of these conducting metal oxides together with Pt catalyst could also facilitate the oxidation process of CO intermediate molecules. Hence, these types of metal have high potential to be used together with platinum as anode electrode.
Carbon support
To improve the utilization of the Pt catalysts, the carbon support is also another useful approach to be used together with Pt. Carbon materials are largely used as catalyst support because of its special properties such as relatively stable in both acid and basic electrolyte, good conductivity, and provide high surface area for dispersion of metal catalyst. It is believed that carbon materials have a strong effect that can influence the electrocatalysts properties such as metal particle size, morphology, metal dispersion, alloyed degree, and stability. Carbon supports can also affect the performance of supported catalysts in fuel cells, such as mass transport and catalyst layer electronic conductivity, electrochemical active area, and metal nanoparticle stability during the operation.
Currently, a great concern of the development in the nanotechnology field, especially carbon nanomaterials synthesis, is to create more stable and active supported catalysts. Support materials of nanoparticles are believed to be the most promising materials for catalytic activity in fuel cells, including the DMFC system. Pt has been traditionally used as nobel catalysts for many fuel cells application [126,127,128]. However, the high cost and low reserve are hindering commercialization of fuel cells and driving researchers to make the utmost of the catalyst. According to this problem, the major effort has been done toward nanoscaling of the catalyst nanoparticles to form more active sites per mass unit. The morphology, structure, and activity of the catalyst, and correspondingly the whole lifetime of a cell, thus strongly depend on the catalyst support [129]. Table 2 shows the preparation, physical properties, performance, and activity of Pt-based supported carbon done by groups of researchers. The details of Pt-based supported carbon will be performed in the following sections:“Graphene Support” to “Carbon Nanocoils”.
Graphene support
Graphene has many extraordinary properties; it exists as a two-dimensional carbon (2-D) form, which is called a crystalline allotrope, one-atom-thick planar flat sheet of sp2 tightly bonded carbon atoms with a thickness of 0.34 nm. Its carbon atoms are packed in a regular atomic-scale chicken wire (hexagonal) pattern [92, 119]. The theoretical specific surface area of graphene is 2630 m 2 g −1 , which is much larger than that of carbon black (typically less than 900 m 2 g −1 ) and carbon nanotubes (100 to 1000 m 2 g −1 ) and similar to that of activated carbon [130]. Graphene has high potential as a metal support [131, 132, 133] [33] due to its high surface area [134] for better catalyst/metal dispersion [135], high electrical conductivity [136], and good thermal properties [137, 138]. Moreover, the functionality of graphene support can be modified by changing it surface structure, and hence contribute to its potential applications, such as in fuel cells, energy storage, electrochemistry, supercapacitors, and batteries [138,139,140,141,142]. Figure 4 illustrates the preparation steps to obtain the graphene nanosheets (GNS), while Fig. 5 shows their TEM images [143]. It can be clearly observed that the thickness of the GO with many typical wrinkles obviously decreases compared to graphite. This can be explained by the presence of the rich oxygen-containing functional groups over the surface of GO [132]. Besides, both resulting GN-900 and GN-900-C contained of a large size of nanosheets structure, but the GN-900-C comprised more transparent than the GN-900.
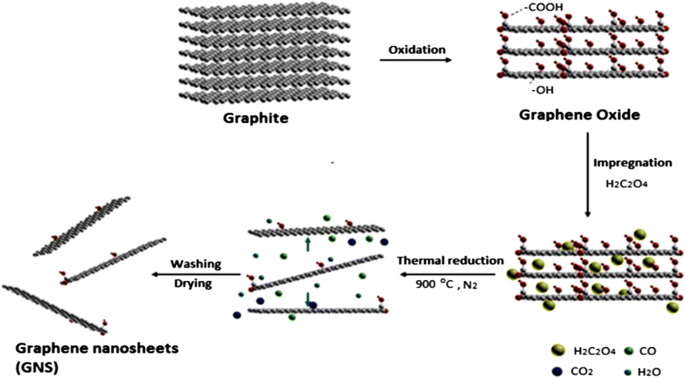
illustration of the preparation of graphite oxide to graphene nanosheets (GNS) by using oxalic acid [143]
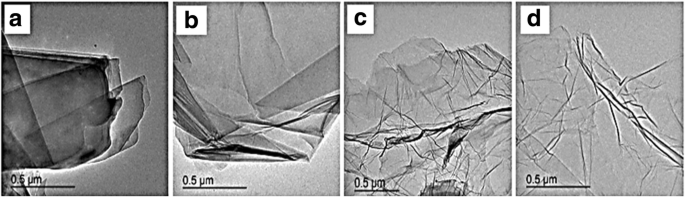
TEM images of graphite (a ), GO (b ), GN-900 (c ), and GN-900-C [143]
The discovery of graphene sheets began around 2000 by mechanical extracting process from 3D graphite source [133]. Graphene can be obtained by several synthesis methods such as hydrothermal [144], chemical reduction [143], chemical vapor deposition, and electrochemical. Ma et al. [145] enhanced the electrocatalytic activity of Pt nanoparticles by supporting the Pt nanoparticles on functionalized graphene for DMFC. Functionalized graphene was prepared by methyl viologen (MV) and Pt/MV–rGO electrocatalyst was synthesized by a facile wet chemical method. They also reported that the higher catalytic activity of Pt/MV–RGO was attributed to the synergetic effect between MV and rGO.
Meanwhile, Zhang et al. [146] modified the graphene support with graphene nanosheets through Hummer’s method, followed by polymerization of aniline (as nitrogen source). The TEM images for Pt/NCL-RGO and Pt/RGO electrocatalysts show that the aggregation between separated graphene sheets was decreased by nitrogen-doped carbon layer (NCL), leading to a better dispersion of the Pt catalyst on the graphene nanosheets support and better electroactivity and stability toward methanol electrooxidation (MOR). Presence of NCL successfully prevented the aggregation of graphene nanosheets as the Pt nanoparticles supporting material.
In 2011, Qiu et al. [135] successfully synthesized nanometer-sized Pt catalyst via sodium borohydride reduction method with an average particle size of only 4.6 nm. These Pt nanoparticles showed an even dispersion of Pt catalyst on graphene oxide support and very high electrocatalytic activity toward MOR by controlling the percent deposition of Pt loaded on the graphene. In another study conducted by Ojani et al. [147], for synthesized Pt-Co/graphene electrocatalyst, it was shown that graphene nanosheets improved the electrocatalytic behavior and long-term stability of the electrode. In addition, the Pt-Co/G/GC electrocatalyst showed great stability toward MOR. The catalytic performance toward MOR can also be improved by using cobalt core–platinum shell nanoparticles supported on surface functionalized graphene [148]. This enhanced catalytic activity could be attributed to the poly (diallyldimethylammonium chloride) (PDDA) that plays a crucial role for dispersion and stabilization of Co@Pt catalyst on graphene support. PDDA-functionalized graphene provided the higher electrochemical active surface area [149, 150]. Huang et al. [138] also studied a PtCo-graphene electrocatalyst with outstanding catalytic performance and high CO tolerance toward the MOR, which far outperformed Pt-graphene and PtCo-MWCNT electrocatalysts with the same ratio of Pt and carbon content. Figure 4 shows the formation of a graphene-PtCo catalyst prepared from a graphite source. Sharma et al. [57] synthesized Pt/reduced graphene oxide (Pt/RGO) electrocatalyst using a microwave-assisted polyol process, which sped up the reduction of GO and formation of Pt nanocrystals. They compared Pt/RGO to a commercial carbon support (Pt/C), which exhibited high CO tolerance, high electrochemically active surface area, and high electrocatalytic activity for the MOR. In a previous study, Zhao et al. [139] reported that the unique 3D-structured Pt/C/graphene aerogel (Pt/C/GA) exhibited greater stability toward MOR with no decrease in electrocatalytic activity. Moreover, the Pt/C/graphene aerogel also exhibited significantly higher stability to scavenge crossover methanol at high potential in an acidic solution compared with the commercial Pt/C electrocatalyst. At the initial catalytic stage, the Pt/C electrocatalyst lost approximately 40% after 1000 CV cycles. In contrast, the Pt/C/graphene aerogel only lost 16% of the initial catalytic activity. After 200 cycles of CV, the current density of Pt/C/graphene aerogel was much higher with a remarkably higher stability than that of Pt/C electrocatalyst. Meanwhile, Yan et al. [151] demonstrated highly active mesoporous graphene-like nanobowls supported Pt catalyst with high surface area of 1091 m 2 g −1 , high pore volume of 2.7 cm 3 g −1 , and average pore diameter of 9.8 nm obtained by applying a template synthesis method. In addition, the Pt/graphene bowls also achieved high performance toward MOR with a current density value of 2075 mA mgPt −1 , which was 2.87 times higher than that of commercial Pt/C (723 mA mgPt −1 ). The onset potential for the Pt/graphene bowls toward methanol electrooxidation was negatively shifted by approximately 160 mV compared with that to the latter and showed CO resistance. Figure 6 shows the proposed schematic for the formation of PtCo catalyst on reduced-GO (rGO) support [51]. It is described that the formation of graphene oxide nanosheets from oxidation of graphite powder leads to the increase in interlayer “d” spacing of stacked graphitic sheets from 0.34 to 0.78 nm due to the presence of various oxygen-containing functional groups. The oxygen-containing functional groups act as anchor sites for the well-dispersed Pt and PtCo nanoparticles on rGO sheets, and used for efficient electrooxidation of methanol.
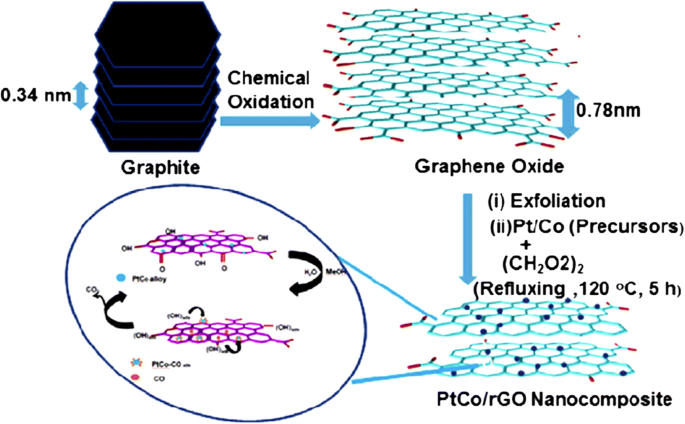
Illustrates the schematic formation of graphene supported Pt-Co catalyst [51]
We can conclude that the reduce graphene oxide (rGO), graphene, modified graphene as supporting material exhibited high electrocatalytic activity toward methanol electrooxidation process. A lot of studies have been reported related to the particle size distribution and size, morphologies, and catalytic activities of Pt and Pt alloys using graphene as supporting material, which showed great improvement in fuel cell performance as mentioned and discussed above. Thus, graphene support can be further studied for better fuel cell performance.
Multiwall Carbon Nanotube and Single-Wall Carbon Nanotube Support
Several years ago, Jha et al. [140] prepared multiwall carbon nanotube (MWCNTs) via chemical vapor deposition using an AB3 alloy hydride catalyst. Platinum-supported MWCNT (Pt/MWCNT) and platinum-ruthenium-supported MWCNT (Pt-Ru/MWCNT) electrocatalysts were prepared by chemical reduction. The performance of these electrodes was studied at different temperatures, and the results demonstrated a very high power density of 39.3 mW cm −2 at a current density of 130 mA cm −2 , which could be attributed to the dispersion and accessibility of the MWCNT support and Pt-Ru in the electrocatalyst mixture for the methanol oxidation reaction. This was also done by other researchers that using different catalyst supported MWCNT for DMFC system [152,153,154,155]. Meanwhile, Wu and Xu [156] compared MWCNT-supported Pt and single-wall carbon nanotube (SWCNT)-supported Pt. Figure 7 shows that the TEM images of Pt catalyst was deposited on MWNT and SWNT electrodes through the electrodeposition technique. The Pt particles in Pt-SWNT (Fig. 7b) looked closer contact with the network of entangled and branched bundles of SWNT support, and the shape is closer to highly exposed sphere. The benefits of the SWCNT support are due to its greater electrochemical surface-active area and easier charge transfer at the electrode/electrolyte interface because of the graphitic crystallinity structure, rich amount of oxygen-containing surface functional groups, and highly mesoporous and unique 3D-structure of SWNT. The electrodeposition technique carried out by them contributed to higher utilization and more uniform dispersion of Pt particles on its support.
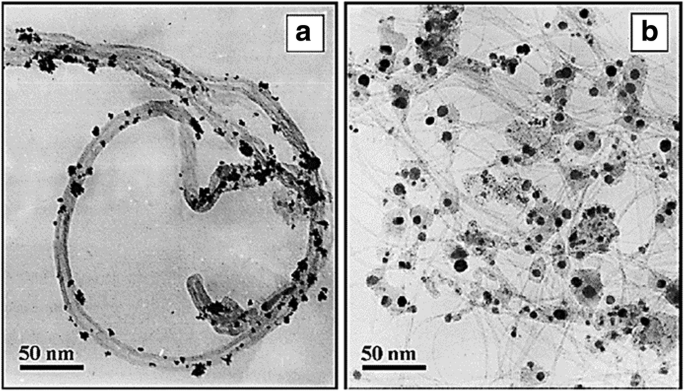
TEM images for the Pt on MWCNT(a ) and SWCNT (b ) [156]
Then, Wang et al. [157] reported the high performance of modified PtAu/MWCNT@TiO2 electrocatalyst prepared via deposition-UV-photoreduction for DMFC, which also exhibited high CO tolerance. Zhao et al. [126] studied 3D flower-like platinum-ruthenium (PtRu) and platinum-ruthenium-nickel (PtRuNi) alloy nanoparticle clusters on MWCNTs prepared via a three-step process, and the best ratios obtained from their experiments for the PtRu and PtRuNi alloys were 8:2 and 8:1:1, respectively. Another group, i.e., Zhao et al. [158], found a higher current density toward MOR and better activity for MWCNT-supported PtWC compared with Pt/C electrocatalyst. These results were attributed to the factors of the synergistic effect between the Pt catalyst and the WC component, high CO tolerance from the bifunctional effect of the Pt catalyst and the WC component, and strong interaction between metals and WC in the electrocatalyst composite.
As a summary, both of MWCNT and SWNT support in terms of structural, surface, and electrochemical properties have their own characteristics as supporting material that remarkably enhanced their performance in catalysis of methanol oxidation process. However, as a comparison, SWCNT possess a high degree of graphitization, highly mesoporous 3D structure, and contain more oxygen-containing functional groups at its surface sites. In relation with these properties, the SWCNT exhibits a higher electrochemically accessible surface area and faster charge transfer rate at the electrode/electrolyte interface.
Carbon Nanotube Support
Wen et al. [144] proposed that carbon nanotubes (CNTs) support could improve fuel cell performance; for example, Pt can be fixed to the inner wall and the outer wall of CNTs and may cause improvement in the electrocatalytic properties of platinum-CNTs. Yoshitake et al. [159] proposed that fuel cells using CNTs as the catalyst support produced larger current densities. The addition of binary or other components to the electrocatalysts for methanol electrooxidation overcomes the problems related to catalyst poisoning caused by CO during the reaction. Therefore, new electrocatalyst carbon supports, such as carbon nanotubes [160, 161], are being actively developed to significantly improve fuel cell performance. Kakati et al. [128] successfully synthesis the PtRu on CNT/SnO2 for anode catalyst DMFC via hydrothermal process. It has been found that the presence of SnO2 provide a high durability property for the catalyst and the presence of SnO2 in the district of Pt could supply oxygen-containing functional groups for the removal of CO intermediate molecules from the Pt surface sites during electrooxidation of methanol. Generally, the decomposition methanol occurs at Pt surface sites; meanwhile, the decomposition of water occurs at SnO2 surface sites to form oxygen-containing species which then react with CO intermediate molecules. However, as support material, the conductivity property of SnO2 still needs to be enhanced. Kakati et al. [128] also proposed the schematic diagram of the formation of PtRu on CNT/SnO2 composite as shows in Fig. 8, and FESEM images of CNT/SnO2 composite support (a and b) and PtRu/SnO2 /CNT composite electrocatalyst (c and d) in Fig. 9.
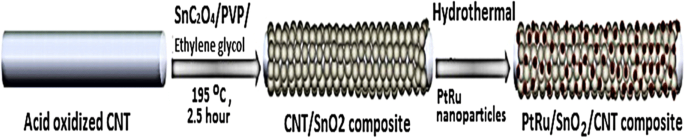
Illustrates the schematic diagram for the formation of PtRu/SnO2/CNT composite [128]
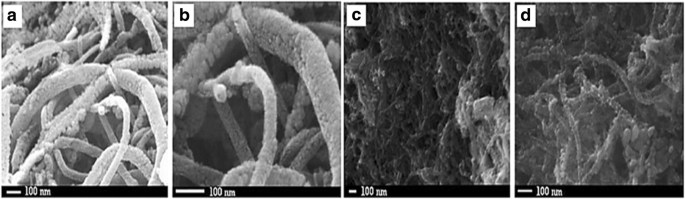
FESEM images of CNT/SnO2 composite support (a , b ) and PtRu/SnO2/CNT composite electrocatalyst (c , d )
Chien et al. [127] proposed that the high catalytic performance of Pt-Ru/CNT for MOR can be attributed to the presence of CNT as the carbon support material with several factors:(i) the as-synthesized Pt-Ru/CNT electrocatalyst owns the ideal nanosized particles and composition to increase catalytic activity, (ii) the presence of functional group on the CNT surface results in high hydrophilicity of CNT, which produces better electrochemical reaction on the electrode area, and (iii) the high electronic conductivity of the CNT support lowers the resistance in MOR. Jeng et al. [150] prepared Pt-Ru/CNT electrocatalyst via a modified polyol with a PtRu composition ratio of 1:1, exhibiting high catalytic activity toward MOR and better performance than that of commercial PtRu/C. Show et al. [162] reported that Pt catalyst with a size of less than 10 nm can be obtained by dispersing the Pt particles on a CNT surface using the in-liquid plasma method, and excellent performance was demonstrated by the electrical power achieving 108 mW cm −2 [162]. The in-liquid plasma method was also used by Matsuda et al. [163] that can applied to obtain nanometer-sized Pt catalyst on support material that remarkably enhanced the fuel cell performance.
To be concluded, high electric conductivity, large surface area, excellent chemical and electrochemical stabilities, quasi one-dimensional structure, and good morphology as the supporting materials are the key factors of carbon nanotubes (CNTs) in enhancing the DMFC performance. In addition, carbon support materials such as CNTs which contribute a large effect on metal distribution and size have also been proven to be an essential to the electrocatalysts to achieve high catalytic activity during methanol oxidation process.
Carbon Nanofiber Support
Steigerwalt et al. [164] reported the successful synthesis of PtRu alloy that was widely dispersed on a graphene carbon nanofiber (CNF) support as an electrocatalyst in DMFC. The catalytic activity was enhanced by ~ 50% relative to that recorded for an unsupported PtRu colloid anode electrocatalyst. Meanwhile, Wang et al. [152] reported that Pt/CNF nanocomposites obtained by the reduction of hexachloroplatinic acid (H2 PtCl6 ) precursor with formic acid (HCOOH) in aqueous solution containing electrospun CNFs at room temperature showed a higher current density than other prepared Pt/CNFs and was approximately 3.5 times greater than that of the E-TEK Pt/C electrocatalyst. Another research carried out by Giorgi et al. [153] described a CNF and bimetallic PtAu electrode with a single layer and both diffusive and catalytic functions using a decreased noble metal amount (approximately five times less) with a consequent large cost reduction. In addition, the bifunctional electrocatalytic properties were also active for the MOR on the PtAu nanoparticle catalysts [154]. Calderón et al. [155] reported PtRu/CNF prepared via reduction using sodium borohydride (NaBH4 ), methanol, and formate ions. This electrocatalyst synthesized by SFM was heat-treated (denoted as SFM TT), which improved its electrocatalytic activity during MOR. Later, Maiyalagan [165] reported that the addition of silicotungstic acid acted as a stabilizer for the PtRu particles on CNT support. The PtRu-supported CNT was prepared by microwave heating of an ethylene glycol (EG) solution of STA, H2 PtCl6 .6H2 O (as Pt precursor), and RuCl3 .xH2 O (as Ru precursor) with CNF suspended in the solution. The Pt and Ru precursors were loaded on CNF by conventional impregnation method. The results revealed that the PtRu nanoparticles are uniformly dispersed on carbon nanofiber support, with an average particle size of 3.9 nm enhanced the catalytic activity toward methanol electrooxidation. As a conclusion, the carbon nanotubes supporting material with high electronic conductivity and high surface area gives an advantage of better dispersion for the Pt or Pt alloys deposition. The higher the surface area of supporting material can reduce the agglomeration of metal particles on it, thus can produce better catalyst morphology for better fuel cell performance.
Mesoporous Carbon Support
Mesoporous carbon (MPC) support is another ideal candidate as an electrocatalyst support material in DMFC and fuel cell. Generally, mesoporous carbons are divided into two classes based on their structures which are ordered mesoporous carbons (OMCs), with highly ordered pore structure and uniform pore size, nonordered mesoporous carbons with irregular pores. Other than that, OPC can be produced by using high quality of SBA-15 silica and sucrose as carbon source template. To prepare the high quality of SBA-15 SBA-15 sample, triblock copolymer, EO20-PO70EO20 (Pluronic P123, BASF), as the surfactant and tetraethyl orthosilicate (TEOS, 98%, Acros) as the silica source are used, as reported by literature [166,167,168]. The synthesis of MPC starts from synthesis of SBA-15, followed by calcination process.
A well-dispersed and ultralow Pt catalyst (PtFe) supported on ordered mesoporous carbon (OMC) was prepared via a simple route and showed superior catalytic activity. The PtFe alloy with a size range of 3–5 nm was homogeneously dispersed on the CMS with a very high specific surface area of more than 1000 m 2 g −1 [169]. The incorporation of Fe was discussed in the previous section (“Performance of Various Types of Pt-Based Catalysts” section and “Performance of Pt-Based Alloys” section). The high specific surface area of mesoporous carbon support can be produced by carbonization process of a resorcinol-formaldehyde polymer with a cationic polyelectrolyte as a soft template [160]. The performance of Pt/MPC also related to the synthesis/preparation method as done by Kuppan and Selvam. Kuppan and Selvam [167] synthesized four type of Pt/mesoporous carbon by using different reducing agent which are NaBH4 , EG, hydrogen, and paraformaldehyde. From there, the Pt/mesoporous carbon synthesized using paraformaldehyde as reducing agent for showed highest current density. The highest in catalytic was attributed to the use of paraformaldehyde that gives the smallest Pt particle size (4.5 nm), and the highest ECSA (84 m 2 /g) belongs to Pt/mesoporous carbon.
Wang et al. [161] synthesized a Pt@WC/OMC electrocatalyst composite, in which the composite was platinized using a pulsed microwave-assisted polyol technique. The OMC produced in this synthesis exhibited high surface area property. The Pt@WC/OMC electrocatalyst also showed high activity, desirable stability, and CO tolerance toward MOR. In another work done by Zhang et al. [170], the ordered CMS had a unique hierarchical nanostructure (with a 3-D structure) with ordered large mesopores and macropores that facilitated the dispersion of Pt nanoparticles and rapid mass transport during the reactions.
To maximize the use of Pt particles, the support materials should have uniform dispersion, high utilization efficiency, and desirable activity and stability. Moreover, the good supporting materials must be suitable for surface chemistry, high loading of Pt dispersion, and some functional roles. Additionally, based on the previous studies as discussed above, the ordered mesoporous carbons with large pore sizes are highly desirable for fast mass transfer and, thus, enhance the catalytic activity especially in the reaction involve large reactants molecules.
Carbon Black
Carbon black (CB) is one of the commercial carbon support that has been used till now. There are many types of CB such as Vulcan XC-72, Black Pearl 2000, Denka Black, Shawinigan Black, Ketjen EC-300J, etc. [171, 172]. CB is commonly used as a carbon support material for electrocatalysts because it possesses high porosity properties, which make it suitable as a potential support material for the catalyst layer in PEMFCs and DMFCs as reported in provided literatures [173,174,175,176,177,178,179,180]. The comparison of the several carbon black support was reported by Wang et al. [181] who investigated the effect on DMFC performance using several types of carbon black such as Vulcan XC-72R, Ketjen Black EC 300J, and Black Pearls 2000 carbon black as additives/support for the Pt cathode catalyst. From the experiments, the results showed that Ketjen Black EC 300J was the most useful carbon support for increasing the electrochemical surface area and DMFC performance of the cathode catalyst.
Nowadays, CB is commercial carbon support for many fuel cell systems. Generally, it is used for the comparison with new or modified catalyst [125]. The following Table 3 summarizes the commercial carbon black and its properties for fuel cell application. There are so many modifications among carbon support materials and development of new carbon support for enhance fuel cell performance; however, commercial carbon black still is used in many fuel cell applications especially for the comparison with new or modified catalyst.
Carbon Nanocoils
Celorrio et al. [182] proposed carbon nanocoils (CNCs) as a PtRu support in their experiment, indicating that the electrocatalyst performance was strongly dependent on the synthesis method. CNC-supported electrocatalysts showed better electrochemical behavior than E-TEK electrocatalysts, and better electrocatalytic behaviors toward CO and methanol oxidation were achieved using CNC as a support material [182]. Sevilla et al. obtained highly graphitic CNCs from the catalytic graphitization of carbon spherules via the hydrothermal treatment of different saccharides which are sucrose, glucose, and starch [183]. They demonstrated that the high electrocatalytic activity of the CNCs is due to the combination of good electrical conductivity of their graphitic structure and high porosity property, which allows much less diffusional resistance of reactants/products. Two years later, Sevilla et al. [184] reported highly dispersed Pt nanoparticles on graphitic CNCs with diameters in the range of 3.0–3.3 nm and a very fine particle size distribution. The electrocatalyst possessed large active Pt surface area (up to 85 m 2 g −1 Pt), high catalytic activity toward MOR (up to 201 A g −1 Pt), and high resistance against oxidation, which was noticeably greater than that of the Pt/Vulcan electrocatalyst. Celorrio et al. [185] obtained Pt/CNC electrocatalysts via the impregnation method, which showed that a combination of Pt and CNCs facilitated the CO oxidation process.
Conductive Polymer Supports
Choi et al. [186] synthesized PtRu alloy nanoparticles with two types of conducting polymers, i.e., poly(N -vinyl carbazole) and poly(9-(4-vinyl-phenyl)carbazole), as the anode electrodes. Electrochemical and DMFC tests showed that these nanocomposite electrocatalysts were beneficial in a DMFC system, but their catalytic performance was still lower than that of a carbon supported electrode. Thus, they suggested that higher electrical conductivity of the polymer and lower catalyst loss are required in nanocomposite electrodes to achieve better performance in a DMFC. Choi et al. [171] and Kim et al. [172] prepared polyaniline (PANi) as a support material for PtRu catalyst in a DMFC system. PANi is a group of conductive polymers with high electronic conductivity and a methanol oxidation current similar to that of carbon-supported PtRu catalyst. Then, Kim et al. [172] conducted catalytic tests to compare PtRu/PANi support with PtRu/carbon support, showing that the enhanced catalytic activity of PtRu/PANi was due to (i) the high electrical conductivity of the polyaniline support, (ii) the increase of electrochemical surface area of the prepared electrocatalyst, and (iii) the higher ion diffusion behavior. In another study, Amani et al. [74] synthesized PtSn supported by C-PANI as an electrocatalyst with different Pt:Sn atomic ratios using the impregnation method. The PtSn/C-PANI electrocatalyst with a ratio of 30:70 showed outstanding performance in the methanol electrooxidation, and the current density was approximately 40% higher than PtRu/C and 50% higher than Pt/C-PANi. The CO tolerance and stability were improved compared to that of PtRu/C, and the methanol crossover was reduced. Yaldagard et al. [173] studied the electrocatalytic performance of Pt/PANi/WC/C electrocatalyst for methanol electrooxidation (MOR) and oxygen electro-reduction (ORR), and it exhibited higher MOR activity, high CO resistance, and improved stability compared to Pt/C electrocatalyst in the presence of methanol.
Wu et al. [174] presented polypyrrole nanowire networks (PPNNs) as the anodic microporous layers (MPLs) of passive DMFC. In passive DMFC system, the novel MPL achieved a 28.3% increase in the power density from 33.9 to 43.5 mW cm −2 compared with the conventional layer with a similar PtRu (1:1). The high performance was due to the presence of PPNNs, which expressively improved the catalyst utilization and mass transfer of methanol on the anode. Besides, Selvaraj and Alagar [175] prepared Pt-Ru nanoparticle-decorated polypyrrole/multiwalled carbon nanotubes (Ppy/CNT) via the in situ polymerization of Ppy on CNTs containing ammonium peroxydisulphate (NH4 )S2 O8 as an oxidizing agent at the temperature range of 0–5 °C, followed by deposition of Pt particles on PPy-CNT composite films via chemical reduction to produce Pt/PPy-CNT. It was found that the PtRu particles deposited on PPy–CNT composite films exhibited higher catalytic activity and stability toward MOR compared to Pt/PPy-CNT. So far, the investigation on polymer as supporting materials is not much as carbon support materials. From aspect as supporting materials, the performance of polymer support was not good/excellent as carbon support. Further studies are needed in the future for better electrocatalytic activity and DMFC performance.
Problems and Limitations of Using Pt for DMFC Systems
There are two major challenges in the development of new DMFC catalysts:(i) performance, including the catalytic activity, reliability, and durability; and (ii) catalyst cost reduction. Two major problems arise in DMFC when using pure Pt alone as the anode catalysts:(1) slower kinetics oxidation of methanol, even on some state-of-the-art anode catalysts, and methanol crossover through the membrane, which not only lowers cathode performance but also reduces fuel efficiency. To develop successful fuel cell technology, including DMFC technology, new catalysts must be investigated to improve the performance and reduce the cost. Reduction of the catalyst cost remains a major challenge. Currently, platinum is one of the most effective electrocatalysts for DMFC due to its high catalytic activity for methanol oxidation, but because it is a precious metal, platinum usage is challenging and limited [176, 177]. Therefore, many scientists have attempted to find materials that can behave like Pt catalysts. One problem with the MOR in DMFCs is that CO is produced as an intermediate reaction product when using Pt catalyst and has strong binding energy on platinum particles, poisoning the active sites of the platinum surface area [58]. Therefore, CO must be removed by oxidizing it from the Pt surface using another material with high resistance to CO poisoning. For example, Hwu et al. proposed Pt-modified WC catalyst that has remarkable resistance to CO poisoning [178]. On the other hand, they also suggested that CO tolerance originates from the lower CO desorption temperature on pure and Pt-modified WC compared to pure Pt.
There are many solutions that can be applied to reduce the cost of Pt, overcome or minimize the formation of CO species during methanol oxidation, and increase the kinetics of methanol oxidation, such as alloying with other metals or transition metals, the incorporation of metals, metal nitrides, and metal oxides and the use of carbon supports as discussed in this paper. However, to overcome this problem, we need to understand the formation of CO on Pt sites particle, and understanding of the mechanism of the anode reaction in DMFCs. Unfortunately, it has limited amount of mechanistic insight to be studied, because this reactions involve complex mechanism path with many possible intermediate molecules and also competing reaction pathways [179]. For Pt catalytic mechanism, it has been suggested by a direct reaction path. Unfortunately, the use of Pt on other metals has limited mechanistic information available. Figure 10 represents the reaction path for methanol electrooxidation and their possible intermediates molecules formed during the process. Black arrows show direct path, while green arrows show the indirect mechanism for CO2 formation as a final product. In the direct mechanism, the reaction path does not involve a CO intermediate, and CO2 is formed directly from methanol. In contrast, indirect mechanism forming a CO intermediate molecule and subsequently it is oxidized to CO2 Product. Notably, CO is the most stable molecule of all the intermediates on Pt during MOR. The stability of CO causes it to be a main reason for the extensive CO poisoning problem that is often found on Pt catalyst.
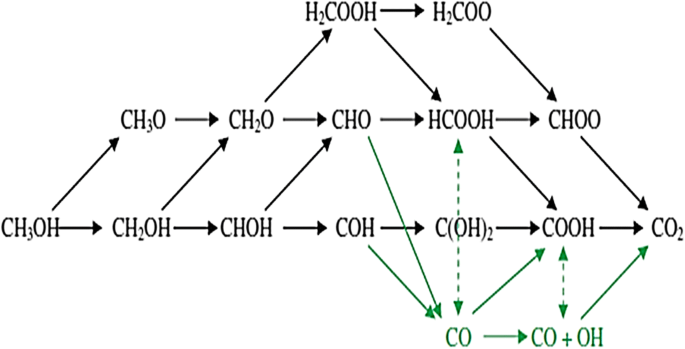
Schematic of the reaction paths and possible intermediates molecules considered in methanol electrooxidation [237]
First step in the mechanism of methanol decomposition reaction on Pt is the activation of methanol molecule. It can take place via hydrogen abstraction from either the carbon or the oxygen atoms. Further step, hydrogen abstraction creates formaldehyde (CH2 O) or hydroxymethylene (CHOH), followed by formyl (CHO) or COH. In the direct mechanism, instead of stripping off the final hydrogen from CHO or COH molecule to CO, a water molecule will release a proton/electron pair and resulting to OH species that can further bind with the carbonaceous species to form dihydroxycarbene (C(OH)2 ) or formic acid (HCOOH). This step is called hydroxyl addition process. The next step is followed by dehydrogenation to form either formate (HCOO) or carboxyl (COOH) molecule, with subsequent dehydrogenation to form CO2 as the final product of reaction. In addition, an alternative direct mechanism involve the stripping of a proton/electron pair from water and addition of the resulting hydroxyl to CH2 O, subsequently to H2 COOH, which then undergoes dehydrogenation to form HCOOH or dioxymethylene (Hs COO). The Hs COO molecule can then undergoes dehydrogenation to HCOO and finally to CO2 . Besides, in the indirect mechanism, CHO or COH species are directly dehydrogenated to CO. Water is dissociated separately on the surface to form OH, and the two surface species react together to form CO2 gas in a way similar to the water-gas-shift reaction [187]. This indirect mechanism occurs because less energy is required to form CO than CO2 . Strong adsorbed CO intermediate form on the Pt surface sites revealed a major problem at the anode site of DMFC. Formation of this intermediate species can cause deactivation Pt catalyst. Furthermore, the rate of kinetic methanol oxidation for DMFC is slower. Therefore, to increase the resistance of Pt catalyst to CO poisoning on the electrodes, Pt alloy or hybrids, such as PtRu, PtSn, PtMO, PtPb, PtFe, PtCo, PtNi, PtRuOs, PtRuMo, PtRuSn, PtRuNi, etc. (as mentioned and discussed in “Performance of various types of Pt-based catalysts” section), are usually employed as electrocatalyst materials on DMFC anodes. Addition/incorporation of these alloys to Pt can prevent the adoption of CO on Pt surface by decreasing the oxidation overpotential of the anode [84].
Conclusion and Prospects
Great progress has been made in recent years in the development and optimization of new catalysts using Pt-based catalysts and carbon and conductive polymer supports for DMFC anode catalyst. Some new carbon materials, such as nano- or mesostructured carbons, have been demonstrated as highly potential catalyst support materials, although their applications face challenges in terms of synthesis, metal loading, and electrode preparation. The combination of platinum as the best metal catalyst for DMFC and an excellent carbon support could produce breakthroughs in the investigation of a new DMFC anode catalyst in the future. Since platinum is an expensive metal, it is necessary to reduce the amount of Pt used in the electrocatalyst. Therefore, this paper presented more than 100 studies on the electrocatalytic activity and performance related to Pt-based electrocatalysts and various carbon and conductive polymer supports. The main problems related to the platinum electrocatalyst, such as carbon monoxide formation during the methanol oxidation reaction and the poor kinetics of methanol oxidation, could be overcome using additional materials and various supports, as reported in the research presented in this paper.
Many studies conducted in the recent years to reduce the loading amount of Pt catalyst and to increase the percentage utilization efficiency, and hence, enhance the electrocatalytic activity of Pt toward the oxygen reduction reaction (ORR) and methanol electrooxidation reaction (MOR), were discussed in this paper. Pt has been alloyed with many transition metals such as Fe, Co, Ni, Ir, Ru, Rh, and Pd, resulting in higher catalytic activity for the DMFC system. The incorporation of these materials also resulted in good dispersion on the carbon and polymer supports, which showed higher performance in the DMFC test compared to the use of Pt metal alone. Various carbon support sources, namely activated carbon (AC), carbon black (CB), multiwall carbon nanotubes (MWCNTs), carbon nanofibers (CNFs), carbon nanotubes (CNTs), graphene, and conductive polymer supports, have been used with Pt-based catalysts to improve their catalytic performance. Additionally, Pt-based alloy catalysts have been designed as hollow mesoporous PtNi, nanowire PtRu, and nanodendritic PtRh, which showed improved electrocatalytic activity and superior electrocatalytic performance. Meanwhile, 3-D Pt/C/graphene aerogel demonstrated enhanced stability toward methanol electrooxidation. The work performed by researchers showed that the electrocatalytic activities of nanoparticles Pt alloy catalysts depend on several factors such as the synthesis method, condition of experiments (such as temperature and pH), alloy composition/ratio, precursors, and thermal treatment. For the future study, it should be extended to the optimization of the geometry and structure of previous studies that revealed active Pt alloys can increase their electrocatalytic activity and stability and the application of support materials for fuel cell applications. For example, current research that have been done by Liu et al. 2017 [188] shows the excellent performance of platinum. From theoretical calculations, it revealed that the main effective sites on platinum single atom electrocatalysts are single-pyridinic-nitrogen-atom-anchored single-platinum-atom centers, which ascribed to the tolerant CO in MOR. They also suggested that carbon black supported used together with Pt single atom is effective in cost, efficient, and durable electrocatalyst for fuel cell application. According to the above study, herein, we can conclude that the modification on structure and morphology of precious metal such as platinum could also remarkably increase the performance of electrocatalyst, but in the same time can reduce the overall cost of fuel cell for commercialization.
To improve the morphologies of Pt and Pt alloys, carbon support material also need further study. Nanoporous metals become an interesting part of catalyst to be studied for fuel cell application. It is determined very suitable for fuel cell catalysts because they possess high surface area, three-dimensional (3D) network structures with adjustable ligament/pore sizes suitable for mass transport, and electron conduction. Around 2017, Li et al. successfully carried out modification on Pt-Pd-Au trimetallic surface as cathode for oxygen reduction reaction [189]. The surface evolution of 3-D Pt-Pd-Au trimetallic greatly enhanced the ORR activity and highly stable as ORR catalyst. The modification of PtNi alloy also done by Li et al. 2016 [190] showed ultrafine jagged platinum nanowire with highly large ECSA that exhibits enhanced mass activity of ~ 50 times higher than state-of-the-art commercial Pt/C catalyst, while Bu et al. 2016 [191] reported highly uniform PtPb/Pt core/shell nanoplate with biaxially strain extremely active, stable for anodic oxidation reactions, and great performance compared to commercial Pt/C in both methanol oxidation reaction (MOR) and ethanol oxidation reaction (EOR). Since the nanostructured platinum becomes an efficient catalyst for fuel cells as well as various industrial chemical reactions. Thus, these modifications on surface of Pt particles electrocatalysts could also to be applied in MOR for future DMFC.
On the other hand, to reduce the consumption of the Pt catalysts, the modification of the carbon support is also another useful way. This not only improves the transport capacity of protons but also reduces the usage of Nafion, which can cut the cost of the fuel cell. Moreover, with regards to the carbon support for the ORR catalysis, the hydrophobic carbon support material is required to allow water (product) to be quickly removed from the catalyst surface sites, and oxygen (reactant) to access the active sites. In contrast, the MOR catalysis requires a certain degree of hydrophilic carbon support. It can be achieved by the modification of the carbon support materials. By combination of modified carbon support materials and development of new carbon support with Pt metal catalyst, it is possible to get an ideal electrocatalysts for direct methanol fuel cell technology. Combination of Pt metal with varied carbon supports with different specific surface areas, structures, pore sizes, electronic properties, and morphologies could be great catalyst to be studied for future DMFC.
Carbon support also influence the overall performance for DMFC. Vulcan XC-72R, which is a commercial carbon support, has a large surface area, appropriate particle size, and good electrical conductivity for good support. However, in the process of depositing metal particle on these support with loading of 40% or more, the particle size of metal increased quickly, which is a disadvantage for DMFC, because a higher metal loading is used to give a better performance. In addition, multiwalled carbon nanotubes (MWCNTs) and carbon nanofibers (CNFs) with relatively smaller surface area, large diameter, and high aspect ratio could be very difficult to deposit a catalyst with high loading metal (40% and more). Therefore, modification of MWCNTs and CNFs support must be done to improve its surface area, surface functional groups, and reduce the wall thickness to achieve outstanding performance for direct methanol fuel cell even though high loading metal catalyst is consumed. As well, a great and important part to be further studied in DMFC system is about the anode and cathode catalyst preparation approaches.
Afkortingen
- CB:
-
Carbon black
- CH3 O:
-
methoxy group
- CNC:
-
Carbon nano cage
- CNF:
-
Carbon nano fiber
- CNT:
-
Carbon nano tube
- Co:
-
Cobalt
- Co:
-
Cobalt
- CO:
-
Monoxide molecules
- CO2 :
-
Carbon dioxide
- DMFC:
-
Direct methanol fuel cell
- FC:
-
Fuel cell
- Fe:
-
Iron
- MOR:
-
Methanol oxidation reaction
- MPC:
-
Mesoporous carbon
- MWCNT:
-
Multi wall carbon nanotube
- Ni:
-
Nickel
- OMC:
-
Ordered mesoporous carbon
- ORR:
-
Oxygen reduction reaction
- PANi:
-
Polyaniline
- PEMFC:
-
Proton exchange membrane fuel cell
- Ppy:
-
Polypyrrole
- Pt:
-
Platinum
- Pt/MWCNT:
-
Platinum-supported MWCNT
- Pt-Ru/MWCNT:
-
Platinum-ruthenium-supported MWCNT
- Rh:
-
Rhodium
- Ru:
-
Ruthenium
- Sn:
-
Sternum
- SOFC:
-
Solid oxide fuel cell
- SWCNT:
-
Single-wall carbon nanotube
- TMN:
-
Transition metal nitride
Nanomaterialen
- Multifunctionele gouden nanodeeltjes voor verbeterde diagnostische en therapeutische toepassingen:een overzicht
- Vooruitgang en uitdagingen van fluorescerende nanomaterialen voor synthese en biomedische toepassingen
- Grafeen- en polymeercomposieten voor toepassingen met supercondensatoren:een recensie
- Vervaardiging en karakterisering van nieuwe composiet Tio2 koolstof nanovezel anodische katalysatorondersteuning voor directe methanolbrandstofcel via elektrospinmethode
- Verbeterde prestaties van een nieuwe anodische PdAu/VGCNF-katalysator voor elektro-oxidatie in een glycerolbrandstofcel
- Evaluatie van grafeen/WO3 en grafeen/CeO x-structuren als elektroden voor supercondensatortoepassingen
- Nieuwe Anodic Catalyst Support voor Direct Methanol Fuel Cell:Karakteriseringen en Single-Cell Performances
- Biomedische toepassingen voor gouden nanoclusters:recente ontwikkelingen en toekomstperspectieven
- Review:poreuze metalen filters en membranen voor olie-waterscheiding
- Fluorescerende neoglycoproteïne gouden nanoclusters:synthese en toepassingen in lectinedetectie bij planten en celbeeldvorming
- Solvay lanceert hoogwaardige koolstofvezeltape voor offshore olie- en gastoepassingen