Nanodeeltjes voor kankertherapie:huidige vooruitgang en uitdagingen
Abstract
Kanker is een van de belangrijkste doodsoorzaken en morbiditeit met een complexe pathofysiologie. Traditionele kankertherapieën omvatten chemotherapie, bestralingstherapie, gerichte therapie en immunotherapie. Beperkingen zoals gebrek aan specificiteit, cytotoxiciteit en resistentie tegen meerdere geneesmiddelen vormen echter een aanzienlijke uitdaging voor een gunstige kankerbehandeling. De komst van nanotechnologie heeft een revolutie teweeggebracht in de arena van kankerdiagnose en -behandeling. Nanodeeltjes (1-100 nm) kunnen worden gebruikt om kanker te behandelen vanwege hun specifieke voordelen, zoals biocompatibiliteit, verminderde toxiciteit, uitstekende stabiliteit, verbeterde permeabiliteit en retentie-effect, en nauwkeurige targeting. Nanodeeltjes worden ingedeeld in verschillende hoofdcategorieën. Het medicijnafgiftesysteem voor nanodeeltjes is bijzonder en maakt gebruik van tumor- en tumoromgevingskenmerken. Nanodeeltjes lossen niet alleen de beperkingen van conventionele kankerbehandeling op, maar overwinnen ook resistentie tegen meerdere geneesmiddelen. Bovendien, naarmate nieuwe multidrug-resistentiemechanismen worden ontrafeld en bestudeerd, worden nanodeeltjes krachtiger onderzocht. Verschillende therapeutische implicaties van nanoformuleringen hebben nieuwe perspectieven gecreëerd voor de behandeling van kanker. Het meeste onderzoek is echter beperkt tot in vivo en in vitro studies, en het aantal goedgekeurde nanodrugs is in de loop der jaren niet veel toegenomen. Deze review bespreekt talrijke soorten nanodeeltjes, richtmechanismen en goedgekeurde nanotherapeutica voor oncologische implicaties bij de behandeling van kanker. Verder vatten we ook het huidige perspectief, de voordelen en uitdagingen in klinische vertaling samen.
Inleiding
Kanker is een algemene term voor een reeks ziekten die worden gekenmerkt door ongecontroleerde, willekeurige celdeling en invasiviteit. Gedurende meerdere jaren zijn uitgebreide inspanningen gericht geweest op het opsporen van verschillende risicofactoren voor kanker. Voor sommige vormen van kanker is de etiologie op invloedrijke wijze in verband gebracht met specifieke omgevingsfactoren (verworven factoren), zoals straling en vervuiling. Een ongezonde levensstijl, zoals een slecht uitgebalanceerd dieet, tabaksgebruik, roken, stress en gebrek aan lichaamsbeweging, heeft echter een sterke invloed op het bepalen van het risico op kanker [1, 2]. Hoewel deze externe factoren zijn erkend als belangrijke oorzaken van kanker, is de betrokkenheid van mutaties van proto-oncogenen, expressiepatronen van tumorsuppressorgenen en de genen die betrokken zijn bij DNA-herstel moeilijk in te schatten. Slechts 5-10% van de kankergevallen is gerelateerd aan erfelijke genetica [3]. Ouder worden is een andere cruciale risicofactor voor kanker en veel individuele soorten kanker.
Kanker is een van de belangrijkste problemen voor de volksgezondheid wereldwijd en is de tweede belangrijkste doodsoorzaak. Volgens de American Cancer Society zal het aantal nieuwe gevallen tegen het einde van 2021 naar verwachting 1,9 miljoen zijn [4]. De conventionele therapeutische benaderingen die worden gebruikt bij de behandeling van kanker omvatten chirurgie, chemotherapie, bestralingstherapie, gerichte therapie, immunotherapie en hormoontherapie [5, 6]. Hoewel chemotherapie en bestralingstherapie over cytostase en cytotoxiciteitscapaciteiten beschikken [7], worden deze benaderingen vaak in verband gebracht met acute bijwerkingen en een hoog risico op recidieven. De meest voorkomende bijwerkingen die worden veroorzaakt door neuropathieën, onderdrukking van het beenmerg, gastro-intestinale en huidaandoeningen, haaruitval en vermoeidheid. Daarnaast zijn er enkele geneesmiddelspecifieke bijwerkingen, zoals antracyclines en bleomycine-geïnduceerde cardiotoxiciteit en pulmonale toxiciteit [8] (Fig. 1).
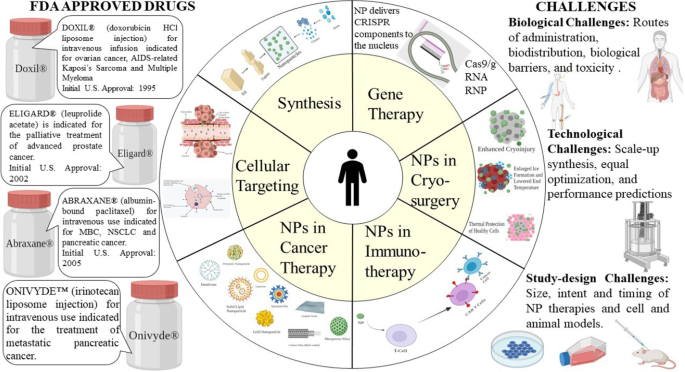
Nanodeeltjes voor kankertherapie
De komst van gerichte therapie heeft geleid tot een groei in precisietherapie [9]. Er zijn echter nog steeds veel onvermijdelijke bijwerkingen, zoals resistentie tegen meerdere geneesmiddelen, waardoor de therapeutische werkzaamheid wordt beperkt [8]. Immunotherapeutische middelen hebben veelbelovende resultaten opgeleverd door niet alleen primaire kanker te behandelen, maar ook door metastasen op afstand te voorkomen en de kans op herhaling te verlagen [10]. Niettemin is auto-immuunziekte een belangrijke bijwerking van immunotherapie. Bovendien suggereren studies en flarden van bewijs dat immunotherapie minder effectief is tegen solide tumoren dan lymfoom [11]. Deze kankers creëren een ongebruikelijke extracellulaire matrix (ECM) die voor immuuncellen behoorlijk uitdagend is om te infiltreren [12]. Deze nieuw ontwikkelde gerichte therapieën en immunotherapieën interfereren met signaalroutes die essentieel zijn bij kwaadaardig gedrag en normale homeostatische functies van de epidermis en dermis en veroorzaken dermatologische bijwerkingen (dAE's) [13].
Gezien al deze details, is de vraag naar de vooruitgang van nieuwe strategieën voor het zoeken naar nauwkeurige therapie van kanker de afgelopen jaren in een stroomversnelling geraakt. Recente inspanningen zijn geleverd om de beperkingen van bestaande therapeutische benaderingen met behulp van nanodeeltjes aan te pakken. Op nanodeeltjes gebaseerde medicijnafgiftesystemen hebben voordelen weerspiegeld in de behandeling en behandeling van kanker door goede farmacokinetiek, nauwkeurige targeting, verminderde bijwerkingen en resistentie tegen geneesmiddelen aan te tonen [14, 15].
Na de vooruitgang van nanotechnologie is een aantal nanotherapeutische geneesmiddelen op de markt gebracht en op grote schaal op de markt gebracht, en sinds 2010 zijn er nog veel meer de klinische fase ingegaan. Nanotherapeutische geneesmiddelen hebben vooruitgang geboekt op het gebied van medicijnafgiftesystemen en anti-tumor multidrug resistentie (MDR) door een kans te bieden op combinatietherapie met geneesmiddelen en remming van resistentiemechanismen tegen geneesmiddelen [16]. De pionierspoging werd gedaan om nanotechnologie toe te passen in de geneeskunde aan de ETH Zürich in de jaren zestig [17]. Deze combinatie is een betere samensmelting gebleken bij de ontwikkeling van verschillende diagnostische apparaten en betere therapieën. Deze review richt zich voornamelijk op basisprincipes van de toepassing van nanotherapeutica, de huidige uitdagingen en beschrijft het pad van toekomstig onderzoek.
Nanodeeltjes
Nanodeeltjes (NP's) worden technisch gedefinieerd als deeltjes met één dimensie kleiner dan 100 nm met unieke eigenschappen die gewoonlijk niet worden aangetroffen in bulkmonsters van hetzelfde materiaal [18]. Afhankelijk van de algemene vorm van het nanodeeltje, kunnen deze worden geclassificeerd als 0D, 1D, 2D of 3D [19]. De basissamenstelling van nanodeeltjes is vrij complex en omvat de oppervlaktelaag, de schillaag en de kern, die in wezen het centrale deel van het NP is en gewoonlijk het NP zelf wordt genoemd [20]. Vanwege hun uitzonderlijke eigenschappen, zoals een hoge oppervlakte:volumeverhouding, ongelijkheid, submicrongrootte en verbeterd richtsysteem, hebben deze materialen veel belang gewonnen in multidisciplinaire gebieden.
NP's blijken een diepe weefselpenetratie te hebben om het verbeterde permeabiliteit en retentie (EPR) effect te vergroten. Bovendien beïnvloeden de oppervlaktekenmerken de biologische beschikbaarheid en halfwaardetijd door effectief de epitheliale fenestratie te kruisen [21]. NP's die zijn gecoat met polyethyleenglycol (PEG), een hydrofiel polymeer, verminderen bijvoorbeeld opsonisatie en omzeilen de klaring van het immuunsysteem [22]. Bovendien is het mogelijk om de afgiftesnelheid van geneesmiddelen of actieve groep te optimaliseren door de kenmerken van deeltjespolymeer te manipuleren. Al met al reguleren de verschillende eigenschappen van NP's hun therapeutisch effect bij de behandeling en behandeling van kanker.
Synthese van NP's
De NP's hebben verschillende vormen, maten en structuren. Om dit te bereiken worden talrijke synthesemethoden toegepast. Deze methoden kunnen grotendeels worden onderverdeeld in twee hoofdgroepen:1) bottom-up benadering en 2) top-down benadering. Deze benaderingen kunnen verder worden ingedeeld in verschillende subklassen op basis van reactieomstandigheden en werking (Fig. 2).
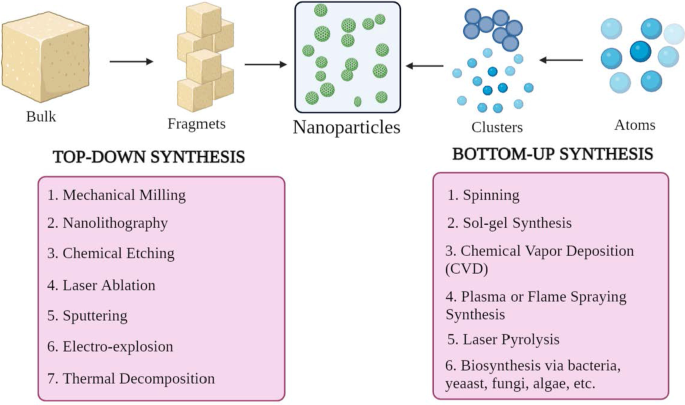
Classificatie van NP-synthese a van boven naar beneden en b bottom-up benaderingen
Bottom-up-benadering
Deze methode omvat bouwmateriaal van atomen tot clusters tot NP's, d.w.z. bouwen van eenvoudigere stoffen, daarom bekend als constructieve methode [23]. Enkele veelgebruikte methoden zijn spinnen, solgelsynthese, chemische dampafzetting (CVD), plasma- of vlamspuitsynthese, laserpyrolyse en biosynthese.
Top-down-benadering
Het is ook bekend als de destructieve methode, die bulkmateriaal of substantie reduceert om NP's te synthetiseren. Een groter molecuul wordt afgebroken of afgebroken tot kleinere eenheden die worden omgezet in NP's [24]. Het omvat technieken zoals mechanisch frezen, nanolithografie, chemisch etsen, laserablatie, sputteren, elektro-explosie en thermische ontleding.
Opmerkelijk is dat de morfologische parameters zoals grootte, vorm en lading van NP's kunnen worden gewijzigd door de reactieomstandigheden en andere syntheseparameters te veranderen [25]. Bovendien bepaalt het groeimechanisme ook de chemische eigenschappen van NP's. Daarom is het begrijpen van het groeimechanisme essentieel om de benodigde NP's te synthetiseren.
Mechanismen van cellulaire targeting
Voor een effectieve kankertherapie is het van essentieel belang om een medicijn- of genafgiftesysteem te ontwikkelen of te manipuleren dat een uitstekend vermogen heeft om zich op tumorcellen te richten en de normale gezonde cellen te sparen. Het verbetert de therapeutische werkzaamheid, waardoor normale cellen worden beschermd tegen het effect van cytotoxiciteit. Het kan worden bereikt door de goed georganiseerde levering van NP's in de tumormicro-omgeving (TME), die zich indirect richt op kankercellen. Deze nanoformuleringen moeten door tal van fysiologische en biologische barrières gaan. Deze barrières zijn complexe systemen van verschillende lagen (epitheel, endotheel en celmembranen) en componenten (mechanische en fysisch-chemische barrières en enzymatische barrières). Deze feiten leggen specificaties op met betrekking tot de grootte, biocompatibiliteit en oppervlaktechemie van NP's om niet-specifieke targeting te voorkomen. Echter, louter cytosolische internalisatie van een NP-medicijnmolecuul betekent niet dat het zijn subcellulaire doelwit bereikt. Specifieke engineering en optimalisatie zijn verplicht om cellulaire of nucleaire targeting mogelijk te maken.
Er zijn tot nu toe verschillende onderzoeken uitgevoerd en er zijn er nog meer aan de gang om op NP gebaseerd ontwerp voor medicijntargeting te ontdekken. Deze nanodragers zouden doorgaans bepaalde fundamentele kenmerken moeten hebben, zoals 1) het vermogen om stabiel te blijven in het vasculaire systeem (bloed) totdat ze hun doel, TME, bereiken, 2) om te ontsnappen aan de reticulo-endotheliale systeem (RES)-klaring, 3) te ontsnappen aan het mononucleaire fagocytsysteem ( MPS), 4) accumuleren in TME via tumorvasculatuur, 5) penetratie onder hoge druk in de tumorvloeistof, en 6) bereiken het doelwit en interageren alleen met tumorcellen [26]. De vitale aspecten zoals oppervlaktefunctionalisering, fysisch-chemische eigenschappen en pathofysiologische kenmerken reguleren het proces van targeting van NP-geneesmiddelen.
Over het algemeen hebben NP's die geschikt worden geacht voor de behandeling van kanker een diameterbereik van 10-100 nm. Om het proces van interactie en overspraak tussen NP-dragers en kankercellen en tumorbiologie te begrijpen, is het belangrijk om de targetingmechanismen aan te pakken. De targetingmechanismen kunnen grofweg in twee groepen worden ingedeeld, passieve targeting en actieve targeting.
Passieve targeting
De waarneming van preferentiële accumulatie van enkele macromoleculen in kankercellen werd eind jaren tachtig gevonden. Het eerste macromolecuul waarvan werd gemeld dat het zich ophoopte in de tumor was poly(styreen-co-maleïnezuur)-neocarzinostatine (SMANCS) door Matsuura en Maeda [27]. Bij verdere studies werd deze preferentiële verdeling toegeschreven aan het optreden van fenestraties die worden gevonden in de beschadigde tumorbloedvaten en aan de slechte lymfedrainage, waarvan de samensmelting bekend staat als "verbeterd permeatie- en retentie-effect".
Onder bepaalde omstandigheden, zoals hypoxie of ontsteking, wordt de endotheellaag van de bloedvaten meer doorlaatbaar [28]. In situaties van hypoxie hebben de snelgroeiende tumorcellen de neiging om meer bloedvaten in werking te stellen of de bestaande te overspoelen om het hoofd te bieden. Dit proces staat bekend als neovascularisatie. Deze nieuwe bloedvaten zijn lekkend omdat ze grote poriën hebben die leiden tot een slechte perm-selectiviteit van tumorbloedvaten in vergelijking met de normale bloedvaten [29, 30]. Deze grote poriën of fenestraties variëren van 200 tot 2000 nm, afhankelijk van het type kanker, TME en lokalisatie [31]. Deze snelle en gebrekkige angiogenese biedt zeer weinig weerstand tegen extravasatie en zorgt ervoor dat NP's uit dergelijke bloedvaten kunnen diffunderen en zich uiteindelijk in kankercellen verzamelen.
In normale weefsels vindt de afvoer van ECF (extracellulaire vloeistof) in lymfevaten vaak plaats met een gemiddelde stroomsnelheid van 0,1-2 µm/s, waardoor de afvoer en vernieuwing constant blijft [32]. Wanneer een tumor wordt gevormd, raakt de lymfefunctie ontspoord, wat resulteert in een minimale opname van interstitiële vloeistof [33]. Deze functie draagt bij aan de retentie van NP's omdat ze niet worden geklaard en opgeslagen in het interstitium van de tumor. Dit proces duidt het verbeterde retentiegedeelte van het EPR-effect aan. Deze uitzonderlijke eigenschap is niet van toepassing op moleculen met een korte circulatietijd en wordt snel uit de kankercellen weggespoeld. Om dergelijke situaties te verbeteren, wordt daarom routinematig het inkapselen van deze kleine moleculen in medicijndragers met nanogrootte uitgevoerd om hun farmacokinetiek te verbeteren, tumorselectiviteit te bieden en bijwerkingen te verminderen [34].
Naast het EPR-effect is TME een essentieel kenmerk bij passieve targeting. Een van de belangrijke metabolische kenmerken van snel prolifererende tumorcellen is glycolyse. Het is de belangrijkste energiebron voor celdeling [35] en maakt de omgeving zuur. Deze verlaagde pH van TME kan worden benut om pH-gevoelige NP's te gebruiken die medicijnen afgeven bij een lage pH [36].
Dit type tumortargeting wordt 'passief' genoemd. Passieve targeting is voornamelijk afhankelijk van verschillende tumorbiologie (vasculariteit, lekkage) en dragerkenmerken (grootte en circulatietijd). Dit type tumortargeting heeft geen specifiek ligand voor bepaalde typen tumorcellen. Het EPR-effect is sterk afhankelijk van de fundamentele tumorbiologie, zoals 1) de mate of omvang van angiogenese en lymfangiogenese, 2) de mate of mate van perivasculaire tumorinvasie, en 3) intratumordruk. Deze factoren, gecombineerd met de fysisch-chemische kenmerken van NP's, bepalen de efficiëntie van het NP-medicijnafgiftesysteem (Fig. 3).
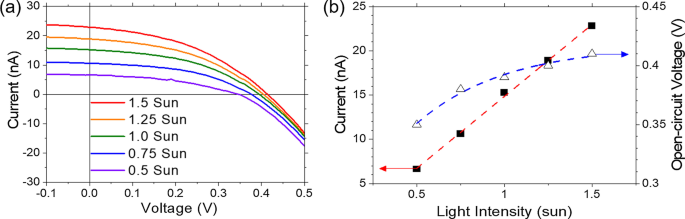
Passieve mobiele targeting
Voorbeelden van passieve targeting
Taxanen zijn een van de meest succesvolle geneesmiddelengroepen die worden gebruikt bij de behandeling van kanker. Paclitaxel heeft een grote potentie getoond tegen een breed scala aan kankers. Borstkanker, longkanker (kleincellig en niet-kleincellig) en eierstokkanker zijn de meest behandelde histologieën met taxanen. De Amerikaanse FDA keurde in 2005 Abraxane® (albumine-gebonden paclitaxel, Abraxis Bio-Sciences) goed, dat wordt gebruikt voor gevorderde of uitgezaaide borstkanker (MBC).
Abraxane® is een geneesmiddel tegen microtubuli dat de microtubuli stabiliseert door depolymerisatie te voorkomen. Het treedt op wanneer het medicijn de microtubuli-assemblage van tubuline-dimeren stimuleert. Deze verkregen stabiliteit belemmert de reorganisatie van microtubuli, wat erg belangrijk is tijdens interfase- en mitotische cellulaire functies. Tijdens de celcyclus en mitose triggert paclitaxel, een veelgebruikt taxaan, respectievelijk ongebruikelijke microtubuli-array samen met meerdere asters. Abraxane® alleen of in combinatie met een ander cytotoxisch middel zoals gemcitabine vermindert pancreasstroma in xenograft-muismodellen van pancreaskanker [37].
Genexol PM® is een innovatieve nanoformulering van paclitaxel en steriele gelyofiliseerde polymere micellaire formulering zonder CrEL. Genexol PM® bleek volgens onderzoeken een driemaal hogere maximaal getolereerde dosis (MTD) te hebben bij naakte muizen. Bovendien vertoonde de biodistributie twee tot drie keer hogere niveaus in verschillende weefsels zoals lever, milt, nier en long en meer prominent in kankercellen. Het is in Zuid-Korea goedgekeurd voor de behandeling van MBC. Het is nog in fase II klinische studie in de VS om alvleesklierkanker te behandelen [38].
DaunoXome® (liposomaal daunorubicine; Gilead Science/Diatos) is een geneesmiddel tegen kanker dat de groei van tumorcellen vermindert. De werkzame stof is daunorubicine. Het is een unieke formulering van daunorubicine (in liposoomvorm) die wordt gebruikt voor de behandeling van Kaposi-sarcoom, een vorm van kanker die de huid, longen en darmen aantast. De Amerikaanse FDA keurde dit in 1996 goed [39].
Hoewel neovascularisatie en angiogenese de NP-diffusie beïnvloeden, leidt dit tot een grotere interstitiële druk, die de accumulatie van NP's remt. Bovendien is de groei van de tumorcellen door de heterogene bloedtoevoer onregelmatig, dat wil zeggen dat de cellen die zich dicht bij de bloedvaten bevinden zich sneller delen dan de cellen die zich verder van het bloedvat bevinden of diep in het kernvormende hypoxische of necrotische gebied binnenin. de tumor. Dit onregelmatige lekken, dat een hoge interstitiële druk veroorzaakt, belemmert de afgifte en accumulatie van geneesmiddelen en vertraagt het neovascularisatieproces [34]. Het is echter mogelijk om het EPR-effect mechanisch of chemisch te beheersen. Deze omvatten stikstofmonoxide, peroxynitraat, bradykinine, VPF (vasculaire permeabiliteitsfactor), echografie, bestraling, hyperthermie, enz. Er zijn echter bepaalde beperkingen en contra-indicaties.
Actieve targeting
Actieve targeting hangt af van specifieke liganden of moleculen, zoals transferrine en folaat, die binden aan moleculen of receptoren die specifiek tot expressie worden gebracht of tot overexpressie worden gebracht op de doelwitcellen (zieke organen, weefsels, cellen of subcellulaire domeinen) [40]. Dit type targeting wordt ligand-gemedieerde targeting genoemd [41]. Hier moeten de NP's die ligand bezitten met specifieke functies zoals retentie en opname in de buurt van het doelwit zijn, zodat er een grotere affiniteit is. Deze strategie verbetert de veranderingen van de binding van NP's aan de kankercel, waardoor de penetratie van geneesmiddelen wordt verbeterd. De belangrijkste aanwijzing hiervoor werd waargenomen in 1980 met antilichamen die in het oppervlak van liposomen werden geënt [34], gevolgd door andere verschillende soorten liganden zoals peptiden, aptameren. Daarom is de belangrijkste methode bedoeld om de overspraak tussen NP's en het doelwit te vergroten zonder de totale biodistributie te fluctueren [42]. Het vitale mechanisme van actieve targeting of ligand-gemedieerde targeting is ligandidentificatie door de doelsubstraatreceptoren. De illustratieve liganden kunnen eiwitten, peptiden, antilichamen, nucleïnezuren, suikers, kleine moleculen zoals vitamines, enz. omvatten [43]. De meest bestudeerde receptoren zijn transferrinereceptor, folaatreceptor, glycoproteïnen en de epidermale groeifactorreceptor (EGFR). Interactie tussen liganden en doelwit leidt tot invouwing van het membraan en internalisatie van NP's via door receptoren gemedieerde endocytose. Er zijn verschillende mechanismen waarmee actieve targeting plaatsvindt. Het merendeel van de tumortargeting wordt gedaan door de tumorceltargeting in het algemeen door NP's. Dit proces verbetert de celpenetratie. Zoals eerder vermeld, is transferrine een van de veel bestudeerde receptoren. Het is een type serumglycoproteïne dat helpt bij het transporteren van ijzer naar cellen. Deze receptoren blijken in de meeste tumorcellen tot overexpressie te worden gebracht, vooral solide tumoren, en komen in lagere niveaus tot expressie in gezonde cellen. Daarom kunnen we de NP's modificeren met geassocieerde liganden die specifiek gericht zijn op transferrine [44]. A2780 ovariumcarcinoomcellen brengen bijvoorbeeld transferrine tot overexpressie. Deze functie wordt gebruikt door met transferrine gemodificeerde PEG-fosfatidyl-ethanolamine (Tf-Mpeg-pe) NP's die specifiek op dergelijke cellen zijn gericht [45]. Een andere alternatieve methode is het richten op cellen naast kankercellen, zoals angiogene endotheelcellen. Deze cellen hebben ook nauw contact met tumorbloedvaten. Deze strategie maakt het mogelijk om hypoxie en necrose te creëren door de bloedtoevoer naar de kankercellen te verminderen. Er is ontdekt dat tumorweefsel zuurder is dan normaal. Dit is uitgebreid verklaard door het Warburg-effect [46]. Dit verklaart de verschuiving van het metabolisme van kankercellen naar glycolyse, waarbij melkzuur wordt gevormd. Wanneer het melkzuur zich ophoopt, sterft de cel af. Om met deze situatie om te gaan, beginnen de cellen protonpompen tot overexpressie te brengen die overtollig melkzuur in de extracellulaire omgeving pompen, waardoor het zuurder wordt. Daarom is een op liposomen gebaseerd pH-gevoelig medicijnafgiftesysteem bestudeerd.
De multivalente aard van de NP's verbetert de overspraak van met ligand gecoate NP's met doelkankercellen. Het ontwerp van dergelijke NP's is complex omdat NP-architectuur en ligand-doelwitchemie de werkzaamheid van de gehele methode beïnvloeden. Andere factoren zoals de toedieningsweg, fysisch-chemische eigenschappen zoals liganddichtheid [47] en grootte van NP's [8] dragen bij aan het succes van het systeem (Fig. 4).
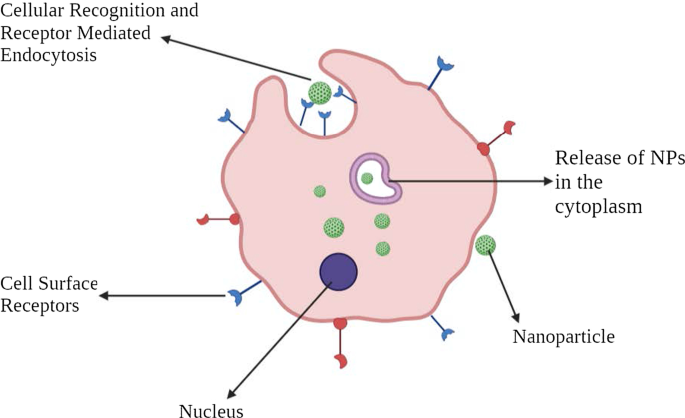
Picturale weergave van actieve cellulaire targeting
Voorbeelden van actieve targeting
EGFR, een lid van de ErbB-familie van tyrosinekinase (TK) -receptoren, wordt tot overexpressie gebracht bij verschillende soorten kanker, vooral bij plaveiselcelhistologie. Gouden NP's met anti-EGFR-PEG-AuNP's en anti-IgG-PEG-Au nanodeeltjes kunnen worden gebruikt om de menselijke SCC aan te pakken [48].
Herceptin® is een therapeutisch medicijn dat zich richt op menselijke EGF-receptor-2 (HER2) die tot overexpressie wordt gebracht op het oppervlak van borstkankercellen. HER2-gerichte gepegyleerde liposomale doxorubicine is ontwikkeld om cardiotoxiciteit te verminderen, een bekende bijwerking van anthracyclines [49].
Het oppervlak van het tumorendotheel brengt een glycoproteïne tot expressie dat bekend staat als vasculaire celadhesiemolecuul-1 (VCAM-1) en dat betrokken is bij het proces van angiogenese. Een onderzoek heeft aangetoond dat NP's zich richten op VCAM-1 in het borstkankermodel, wat wijst op de mogelijke rol ervan [50].
Foliumzuur, ook bekend als vitamine B9, is van vitaal belang bij de synthese van nucleotiden. Foliumzuur wordt geïnternaliseerd door de folaatreceptor die op de cellen tot expressie wordt gebracht. Tumorcellen brengen FR-α echter tot overexpressie (alfa-isovorm van folaatreceptor), terwijl FR-β tot overexpressie wordt gebracht in vloeibare kankercellen [51]. Het richten op de folaatreceptoren door NP's is momenteel voor specifieke kankerbehandelingen [52, 53].
Nanodeeltjes in kankertherapie
NP's die veel worden gebruikt in medicijnafgiftesystemen omvatten organische NP's, anorganische NP's en hybride NP's (Fig. 5).
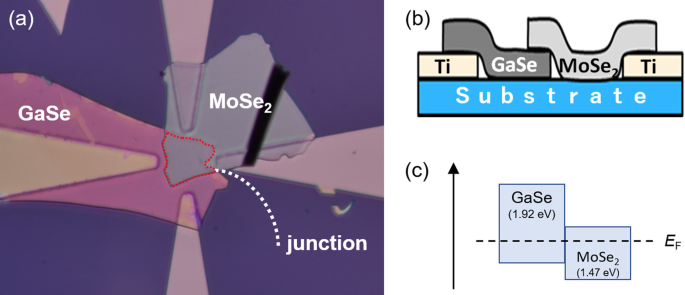
Verschillende soorten nanomaterialen gebruikt bij kankertherapie
Organische nanodeeltjes
Polymere nanodeeltjes
Polymere nanodeeltjes (PNP's) zijn goed gedefinieerd als "colloïdale macromoleculen" met een specifieke structurele architectuur gevormd door verschillende monomeren [54]. Het medicijn is ofwel ingesloten of gehecht aan de buitenkant van NP's, waardoor een nanosfeer of een nanocapsule wordt gecreëerd om gereguleerde medicijnafgifte in het doelwit te bereiken [55]. Aanvankelijk bestonden PNP's uit niet-biologisch afbreekbare polymeren zoals polyacrylamide, polymethylmethacrylaat (PMMA) en polystyreen [56]. De accumulatie hiervan leidde echter tot toxiciteit vanwege de moeilijkheid om deze uit het systeem te verwijderen. Biologisch afbreekbare polymeren zoals polymelkzuur, poly(aminozuren), chitosan, alginaat en albumine worden nu gebruikt en het is bekend dat ze de toxiciteit verminderen en de geneesmiddelafgifte en biocompatibiliteit verbeteren [57]. Bewezen onderzoek heeft aangetoond dat door PNP's te coaten met polysorbaten en door het gebruik van oppervlakteactieve polysorbaten. Exterieurcoating verbetert de interacties van NP's met het endotheelcelmembraan van de bloed-hersenbarrière (BBB) [58].
Een onderzoek toonde aan dat nanocapsules geladen met indomethacine een substantiële afname van de tumorgrootte en verbeterde overleving in een xenotransplantaat glioommodel bij ratten [59]. Dit is een groeiend veld met meer dan tien polymere NP's die geneesmiddelen tegen kanker bevatten die in klinische ontwikkeling zijn. Enkele voorbeelden zijn paclitaxel poliglumex (Xyotax), PEG-camptothecine (Prothecan), gemodificeerd dextran-camptothecine (DE 310), HPMA-copolymeer-DACH-platinaat (AP5346), HPMA-copolymeer-platinaat (AP 5280), HPMA-copolymeer-paclitaxel ( PNU166945) en HPMA-copolymeer-doxorubicine-galactosamine (PK2) [60].
Dendrimeren
Dendrimeren zijn bolvormige polymere macromoleculen met een gedefinieerde hypervertakte architectuur. Sterk vertakte structuren zijn het karakteristieke kenmerk van dendrimeren. Typisch wordt de synthese van dendrimeren geïnitieerd door een ammoniakkern te laten reageren met acrylzuur. Deze reactie resulteert in de vorming van een "trizuur" -molecuul dat verder reageert met ethyleendiamine om "tri-amine", een GO-product, te verkrijgen. Dit product reageert verder met acrylzuur om hexa-zuur te vormen, dat verder "hexa-amine" (Generatie 1) product produceert, enzovoort [61]. Meestal varieert de grootte van de dendrimeren van 1-10 nm. De grootte kan echter oplopen tot 15 nm [62]. Gezien hun specifieke structuur, zoals gedefinieerd molecuulgewicht, instelbare vertakkingen, biologische beschikbaarheid en lading, worden deze gebruikt om nucleïnezuren te targeten. Enkele veelgebruikte dendrimeren zijn polyamidoamine (PAMAM), PEG (poly(ethyleenglycol)), PPI (polypropyleenimine) en TEA (triethanolamine) [63].
Een PAMAM-dendrimeer werd oorspronkelijk ontworpen om MDR-beheer te bereiken. DNA-geassembleerde PAMAM-dendrimeren zijn uitgebreid beschreven. In vergelijking met dieren die werden behandeld met chemotherapie met een enkel middel, vertraagden de gesynthetiseerde dendrimeren de groei van xenotransplantaten van epitheelkanker [64].
mAb-nanodeeltjes
Monoklonale antilichamen worden veel gebruikt bij de behandeling van kanker vanwege hun specifieke doelgerichtheid [65]. Deze mAb's worden nu gecombineerd met NP's om antilichaam-geneesmiddelconjugaten (ADC's) te vormen. Het is bewezen dat deze zeer specifiek en dwingend zijn dan cytotoxische geneesmiddelen of alleen mAb. Een antilichaam-geneesmiddel NP bestaande uit een paclitaxel-kern en een met trastuzumab gemodificeerd oppervlak vertoonde bijvoorbeeld een betere anti-tumor werkzaamheid en lagere toxiciteit dan paclitaxel of trastuzumab alleen in HER2-positieve borstepitheelcelcontrole [66].
Extracellulaire blaasjes
Extracellulaire blaasjes (EV's) zijn dubbellaagse fosfor-lipide blaasjes met een grootte van 50-1000 nm [67]. EV's worden continu uitgescheiden door verschillende celtypen en variëren in oorsprong, grootte en samenstelling. EV's zijn onderverdeeld in drie klassen:1) exosomen, 2) microvesikels en 3) apoptotische lichamen [68]. NP's in combinatie met exosomen worden veel gebruikt omdat ze lipiden en moleculen hebben die erg lijken op oorspronkelijke cellen. Bovendien ontsnappen ze aan de immuunsurveillance en internaliseren ze zich zeer snel in de kankercellen. Ze fungeren als natuurlijke vehikels door cytotoxische geneesmiddelen en andere antitumormiddelen aan de doelplaatsen af te leveren. Exosomen geladen met doxorubicine (exoDOX) zijn het beste voorbeeld. exoDOX wordt gebruikt voor de behandeling van borstkanker en heeft geweldige resultaten laten zien in vergelijking met conservatieve behandeling met doxorubicine door de cytotoxiciteit te verhogen en cardiotoxiciteit te vermijden [69]. Exosome NP's hebben intrinsieke biocompatibiliteitskenmerken, geavanceerde chemische stabiliteit en intracellulaire communicatie in vergelijking met synthetische NP's. Desalniettemin zijn nadelen zoals het ontbreken van standaardomstandigheden voor exosomale isolatie en zuivering cruciaal en moeten worden aangepakt [70, 71].
Liposomen
Dit zijn bolvormige blaasjes die fosfolipiden bevatten die ofwel uni-lamellair of multi-lamellair kunnen zijn om geneesmiddelmoleculen in te kapselen [72]. Liposomen zijn uniek omdat ze kenmerken hebben zoals lage intrinsieke toxiciteit, zwakke immunogeniciteit en biologische inertie [73]. Liposomen zijn het eerste medicijn op nanoschaal dat in 1965 werd goedgekeurd [74]. Een typische liposoomstructuur is samengesteld uit een "hydrofiele kern" en een "hydrofobe fosfolipide dubbellaag". Deze unieke architectuur maakt het voor hen mogelijk om zowel hydrofiele als hydrofobe medicijnen in te sluiten om het ingesloten medicijn effectief te beschermen tegen aantasting van het milieu in omloop [75].
Liposomen bieden ook een uitstekend platform voor medicijnafgifte zoals doxorubicine, paclitaxel en nucleïnezuur door een hogere antitumorwerking en verbeterde biologische beschikbaarheid aan te tonen [76]. Doxil® en Myocet® zijn goedgekeurde op liposomen gebaseerde formuleringen van daunorubicine die worden gebruikt voor de behandeling van MBC [77, 78]. Vanwege tekortkomingen zoals verminderde inkapselingsefficiëntie, snelle verwijdering door MP, celadsorptie en korte houdbaarheid, is de toepassing van op liposomen gebaseerde NP's echter beperkt.
Solid Lipid Nanodeeltjes (SLN)
Het zijn colloïdale nanodragers (1-100 nm) bestaande uit een fosfolipide-monolaag, emulgator en water [79]. Deze staan bekend als nuldimensionale nanomaterialen. De lipidecomponent kan triglyceriden, vetzuren, wassen, steroïden en gePEGyleerde lipiden zijn [80]. Unlike conventional liposomes, SLNs have a “micelle-like structure” within which the drug is entrapped in a non-aqueous core. Examples include mitoxantrone-loaded SLN, which has shown reduced toxicity and enhanced bioavailability [81]. The incorporation of doxorubicin and idarubicin by SLN in “P388/ADR leukemia cells” and the “murine leukemia mouse model” has shown positive results [82].
Nanoemulsions
Nanoemulsions are colloidal NPs with heterogeneous mixtures of an oil droplet in aqueous media ranging from 10–1000 nm [83]. Three representative types of nanoemulsions can be made in:1) oil-in-water system, 2) water-in-oil system, and 3) bi-continuous nanoemulsions. Membrane-modified nanoemulsions have been extensively studied. For instance, nanoemulsions loaded with spirulina and paclitaxel showed an improved anti-tumor effect by regulating immunity through TLR4/NF-kB signaling pathways [84]. Nanoemulsion consisting of rapamycin, bevacizumab, and temozolomide is known to treat advanced melanoma [85]. Nanoemulsions are different from liposomes and certainly have enhanced characteristics than others, such as optical clarity, stability, and biodegradability [86]. However, there are challenges to clinical applications of these nanoemulsions as these involve high temperature and pressure and instruments such as homogenizers and microfluidizers that are expensive.
Cyclodextrin Nanosponges
Cyclodextrins are usually used as stabilizers to increase the drug loading capacity of NPs [87]. Nanosponges are tiny, mesh-like structures [88]. Β-cyclodextrin nanosponges loaded with paclitaxel have shown sound cytotoxic effects in MCF-7 cell line culture [89]. Similarly, camptothecin has shown improved solubility and stability when formulated with cyclodextrin-based nanosponges [90].
Inorganic Nanoparticles
Carbon Nanoparticles
Carbon NPs as the name suggests are based on the element carbon. They have been widely utilized in medical arenas because of their optical, mechanical, and electronic properties combined with biocompatibility [91]. Due to their inherent hydrophobic nature, carbon NPs can encapsulate drugs through π-π stacking [92]. Carbon NPs are further categorized into graphene, carbon nanotubes, fullerenes, carbon nanohorns, and graphyne. Although all these are carbon-based, they vary in their structure, morphology, and properties.
“Graphene” is 2D crystal with sp2-hybridized carbon sheet that holds extraordinary mechanical, electrochemical, and high drug loading properties. Further, based on composition, properties, and composition, graphene can be divided as follows:1) single-layer graphene, 2) graphene oxide (GO), 3) reduced graphene oxide (rGO), and 4) multi-layer graphene [93]. GO and rGOs are widely used due to their ability to target hypoxia [94] and irregular angiogenesis in TME [95]. Studies have shown that GO-doxorubicin exhibits higher anticancer activities in cellular models of breast cancer [96].
Fullerenes are large carbon-cage molecules composed of carbon allotrope with different conformation types such as sphere, ellipsoid, or tube. They are the most widely studied nanocarriers as they have typical structural, physical, chemical, and electrical properties [97]. These are used in photodynamic therapy as they have triple yield and generate oxygen species due to the presence of extended π-conjugation and the ability to absorb light [98]. PEG-modified fullerenes showed promising photodynamic effects on tumor cells [99].
Carbon nanotubes (CNTs) are cylindrical tubes, most often considered as rolls of graphene, were discovered in the late 1980s. They are classified into two groups:1) single-walled CNTs and 2) multi-walled CNTs. As they are carbon-based, they can bring upon immune response by interacting with immune cells, thereby suppressing the tumor growth. Traditionally, they have been used as DNA delivery vectors and for thermal ablation therapy. For instance, a fluorescent single-walled CNT with mAb encapsulating doxorubicin is used to target colon cancer cells. Such CNTs form a complex which is effectively engulfed by the cancer cells leading to the intracellular release of doxorubicin, whereas the CNTs are retained in the cytoplasm [100].
Quantum Dots
Quantum dots are typically nanometer-scale semiconductors with a broad spectrum of absorption, narrow emission bands, and high photostability, allowing them to be widely used in biological imaging [101]. Based on carbon, these are divided into:1) graphene quantum dots, 2) nanodiamond quantum dots, and 3) carbon quantum dots. Besides biological imaging, quantum dots are being actively investigated in cancer treatment. The most commonly used quantum dots is graphene quantum dots due to their inherent biocompatibility and rapid excretion. For example, quantum dots aptamer—doxorubicin conjugate targets prostate cancer cells [102]. However, the deficiency of optimized process in producing quantum dots is the major obstacle.
Metallic Nanoparticles
Metallic nanoparticles are commonly explored in “biological imaging” and targeted DDS due to their remarkable optical, magnetic, and photothermal properties. Some of the most commonly used metallic NPs are gold NPs, silver NPs, iron-based NPs, and copper NPs. Gold NPs are used as intracellular targeting drug carriers because the size and surface properties are easily controlled [103]. Moreover, their visible light extinction behavior makes it possible to track NP trajectories in the cells. “Anti-HER2 functionalized gold-on-silica nanoshells” have been shown to aim HER2 positive breast cancer cells [104]. Combidex®, an iron oxide NP formulation, is presently in the late-stage clinical testing phase to detect nodal metastases [105]. Feraheme®, a ferumoxytol containing iron oxide NP formulation, is used to treat iron-deficiency anemia. This is also used to treat nodal metastases in prostate and testicular cancer and was approved by FDA in June 2009 [106, 107].
Magnetic Nanoparticles
Magnetic NPs are generally used in MRI imaging, and drug delivery contains metal or metal oxides. These are usually covered with organic substances like polymers and fatty acids to enhance stability and biocompatibility [108]. LHRH-conjugated superparamagnetic iron oxide NPs are effective in targeting and imaging of breast cancer [109]. Moreover, magnetic NPs are used in magnetic hyperthermia for thermal ablation of cancer cells [110, 111]. Some of the magnetic NPs that are in the market or in the clinical trial phase are Feridex® and Resovist® for liver metastasis and colon cancer [112].
Calcium Phosphate Nanoparticles
“Calcium phosphate NPs” is biologically compatible, biodegradable, and do not cause any harsh adverse reactions. Hence, they are used as a delivery agent for insulin, growth factors, antibiotics, and contraceptives [113]. They are also used in the delivery of oligonucleotides and plasmid DNA [114]. Calcium phosphate NPs combined with either viral or non-viral vector has been positively used as delivery vectors in cellular gene transfer. A “liposomal nanolipoplex formulation” of calcium and glycerol has shown decreased toxicity and enhanced transfection features [115, 116].
Silica Nanoparticles
Silica being a significant component of many natural materials was only studied concerning biology recently. Silica NPs are commonly used to deliver genes by functionalizing the NP surface with amino-silicanes [117]. N-(6–aminohexyl)–3–aminopropyl–trimethoxysilane functionalized silica NPs have shown excellent efficiency in the transfection of Cos-1 cells with minimal toxicity and is now commercially available [118]. Mesoporous silica NPs are considered one of the best drug carriers due to their better pharmacokinetic properties. They have been extensively used in immunotherapy. According to a study, colorectal cancer cells have shown successful uptake of camptothecin-loaded mesoporous silica NPs.
Mechanism of NPs in Overcoming Drug Resistance
Drug resistance is one of the chief problems in cancer therapy and management. It prevails across all types of cancer and all possible treatment modalities. Drug resistance is a phenomenon that results when diseases become tolerant to pharmaceutical treatments. Drug resistance can be classified into two types:1) innate and 2) acquired [119]. Innate resistance usually results from pre-existing mutations in the genes that are involved in cell growth or apoptosis. Acquired resistance is defined as the type of resistance that is developed after a particular anti-tumor treatment, which may result from the development of new mutations or from alterations in the TME during treatment. Nanoparticles, due to their extraordinary ability to co-encapsulate multiple therapeutic agents, can also be used to overcome cancer-related drug resistance.
Targeting Efflux Transporters
Efflux transporters are classified under the family of “ATP-binding cassette (ABC) transporters.” These have a significant role in MDR. The primary function of these transporters is to pump out drugs out of the cell and reduce the concentration. “P-glycoprotein (P-gp)” is one such efflux transporter that is overexpressed by drug-resistant cancer cells [120, 121].
Overexpression of P-gp has been linked with inadequate treatment response, especially in breast cancer [122] and ovarian cancer [123]. NPs can be used to tackle efflux pumps. As NPs internalize the cell via “endocytosis” instead of diffusion and release the drug at the “perinuclear site,” which is distant from active efflux pumps, NPs can bypass the efflux pumps [124]. Besides, by modifying the control of drug releases, such as by utilizing low pH levels and redox as triggers, NPs can effectively bypass efflux pumps [125, 126].
Combination therapy is yet another method to overcome MDR. NPs can be loaded with multiple drugs within a single drug carrier [127]. Inhibiting efflux transporter expression instead of just dodging them would be another viable option. This can be achieved by building NPs in such a way that it can entrap both efflux pump inhibitors and chemotherapy agents [128]. A recent study positively reflected upon reversing MDR in breast cancer cells by using NPs that co-deliver COX-2 inhibitors and doxorubicin [129]. Similarly, using silica NP that encapsulates miRNA-495 and doxorubicin has proved effective in overcoming drug resistance in lung cancer cells [130]. Another interesting study found out that using NPs in the tumor neo-vasculature targeting KDR receptors is a more effective anti-tumor function than P-gp inhibitor combination therapy. Yet, another way of overcoming drug resistance is by depleting the source of ATP, which is essential for the functioning of ABC transporters. This can be done by targeting mitochondria which leads to a decrease in ATP production.
Targeting an Apoptotic Pathway
Cancer cells proliferate due to faulty apoptotic machinery and upsurge their survival adding to drug resistance [131]. The faulty apoptotic pathway gets activated by “deregulation of Bcl-2” and “nuclear factor kappa B (NF-κB).” These are the most widely investigated anti-apoptotic proteins and can be potentially used as the target for reversing drug resistance. Using a classic process of co-delivery of “Bcl-2 siRNA and chemotherapeutics” by NPs is a way to overcome MDR [132]. NF-κB inhibitors have been used in combination with “pyrrolidine dithiocarbamate (PDTC)” [133] and curcumin [134]. Besides suppressing anti-apoptotic factors, triggering pro-apoptotic factors is another to fight “apoptotic pathway-mediated drug resistance.” For instance, a combination of ceramide and paclitaxel is a good example [135]. Ceramide restores the expression of a chief tumor suppressor, p53 protein, by regulating alternative pre-mRNA splicing. Delivering ceramide via NPs is an excellent way to correct the p53 missense mutation [136]. Owing to its potential, a combination of ceramide and paclitaxel has shown significant therapeutic efficacy in cancer drug resistance models. Transfecting the p53 gene by cationic SLNs has been reported in lung cancer cases [137]. Similarly, transfecting the p53 gene by PLGA has been carried out in breast cancer cells models that have shown potent induction of apoptosis and inhibition of tumor growth [138].
Some NP-based DDS act by impeding efflux pumps and encouraging apoptosis [139]. A pioneering study conducted to prove both pump- and non-pump-mediated drug resistance used an “amphiphilic cationic NP” entrapping paclitaxel and Bcl-2 converter gene in drug-resistant liver cancer models. NP complex diminished P-gp-induced drug efflux and the apoptosis activation. Similarly, co-delivery of “doxorubicin and resveratrol encapsulated in NPs” has shown noteworthy cellular toxicity on doxorubicin resistance breast cancer cells by downregulating the expression of Bcl-2 and NF-κB, thereby initiating apoptosis as well as through the inhibition of efflux transporter expression [140]. A similar study was done on multi-drug resistant prostate cancer cells by using folic acid-conjugated planetary ball milled NPs encapsulated with resveratrol and docetaxel. This worked by downregulating anti-apoptotic gene expression while inhibiting ABC transporter markers [141].
Targeting Hypoxia
Hypoxia is yet an additional aspect that backs MDR [142]. Due to abnormal blood vessels in the vicinity of the tumor and due to the increasing demand of oxygen by the rapidly growing tumor, some tumor cells are repeatedly in a hypoxic condition. The part of the tumor that is in hypoxic condition often escapes from the chemotherapy drugs. Hypoxia creates an oxygen ramp inside the tumor that intensifies tumor heterogeneity, encouraging a more aggressive phenotype. Moreover, the hypoxia condition has been established to facilitate the overexpression of efflux proteins [143]. The major protein, “hypoxia-inducible factor 1α (HIF-1α)” acts an important role. Hence targeting HIF-1α or silencing HIF-1α gene is a way to overcome drug resistance. NPs containing HIF-1α siRNA can be used to reduce hypoxia-mediated drug resistance [144]. Instead of directly targeting HIF-1α, indirect inhibition of HIF-1α signaling can be used. For example, the “PI3K/Akt/mTOR pathway” is known to control the expression of HIF-1α. Inhibition of this pathway effectively downregulates the expression of HIF-1α, which enhances the sensitivity of MDR cells to cancer treatment [145]. NPs like PLGA-PEG and PEGylated and non-PEGylated liposomes can be used effectively. In addition, “heat shock protein 90 (HSP90)” is needed for transcriptional activity of HIF-1 and inhibition of HSP90, which downregulates the expression of HIF-1α [146]. The HSP90 inhibitor in “17AAG loaded NPs” has dramatically improved MDR in bladder cancer treatment [147].
Nanoparticles and Proteomics
When NPs are subjected to the biological system, they are surrounded by cellular and serum proteins which form a structure known as protein corona (PC) [148]. Based on the degree of interaction of these proteins with the NPs, there are classified into the hard corona and soft corona. “Hard corona” is formed when these proteins have a high binding affinity towards the NPs. “Soft corona” is produced when these proteins are loosely bound to the NPS. It has been established that the most protein forming a PC first will be eventually substituted by proteins with higher affinities. This is known as Vroman effect [149]. Hence developing the technology that can manufacture NPs with desired properties is essential. Several proteomic approaches such as MS, LC–MS, SDS-PAGE, isothermal microcalorimetry (ITC), etc. [150], are being used. PC affects the crosstalk of NP with the biological setting and thereby governs the application and usage of the same in the medical field.
Cancer proteomics studies the number of proteins in cancer cells and serum, which supports hunting proteins and biomarkers that aids in diagnosis, treatment, and prognosis [151]. It also helps in understanding cancer pathogenesis and drug resistance mechanism. Post-translational modifications (PTMs) play an indispensable part in occurrence, recurrence, and metastasis. Besides using chemotherapy and kinase inhibitors, novel agents like siRNA, mRNA, and gene editing are central therapeutics used with NPs.
Nanotechnology for Small Interfering RNA (siRNA) Delivery
siRNAs are small ds RNA molecules (around 21 nucleotides long) that suppress the expression of genes in the target. This process is known as “RNA interference.” A few siRNA-based NPs that are currently under clinical investigations are ALN-TTR01 that is used to target the transthyretin gene to treat transthyretin-mediated amyloidosis, and Atu027, which is a liposomal siRNA that targets protein kinase N3 and TKM-ApoB that knock downs the expression of ApoB [152, 153].
Nanotechnology for Tumor microRNA Profiling and Delivery
MicroRNAs are a class of endogenous “single-stranded non-coding RNA” molecules that control post-transcription gene expression by blocking translation of the target mRNA or repressing protein production by destabilizing mRNA [154]. These are emerging as vital biomarkers that are a significant target for cancer diagnosis, therapy, and treatment. The base priming nature of nucleic acid forms the very foundation for nanotechnology used miRNA profiling techniques. Several profiling techniques use biosensors or surface plasmon resonance imaging techniques in combination with molecular biology enzymatic reactions. Nanotechnology can be used for the delivery of MicroRNAs. For example, biodegradable polycationic prodrugs showed promising results in the regulation of polyamine metabolism [155]. MicroRNA-loaded polycation-hyaluronic acid NPs of single-chain antibody fragments have shown progressive downregulation of “survivin expression” in high metastatic cancer load in the lung of murine B16F10 melanoma.
DNA Nanotechnology for Cancer Therapy
DNA-based nanostructures have been synthesized for DNA sensors to detect nucleic acid, DNA-coated gold NPs for lead sensing by hybridizing Pb-activated DNAzyme to the linking DNA, scaffolds to organize organics, inorganic, and biomolecules into distinct morphology molecular transporters, and drug delivery (Table 1).
Advantages of Nanoparticles in Cancer Therapy
The utilization of nanotechnology in the diagnosis, treatment, and management of cancer has led to a whole new era. NPs, either by active or passive targeting, augment the intracellular concentration of drugs while avoiding toxicity in the healthy tissue. The targeted NPs can be designed and altered as either pH-sensitive or temperature-sensitive to establish and regulate the drug release. The pH-sensitive drug delivery system can deliver drugs within the acidic TME. Similarly, the temperature-sensitive NPs release the drugs in the target site due to changes in temperature brought in by sources like magnetic fields and ultrasound waves. In addition, the “physicochemical characteristics” of NPs, such as shape, size, molecular mass, and surface chemistry, have a significant part in the targeted drug delivery system. Further, NPs can be modified according to the target and used to target a particular moiety.
Conventional chemotherapy and radiation therapy have several disadvantages concerning efficacy and side effects because of uneven dispersal and cytotoxicity. Therefore, cautious dosing is required that effectively kills cancer cells without any significant toxicity. To reach the target site, the drug has to pass several fortifications. Drug metabolism is a very complex process. In physiological conditions, the drug needs to pass TME, RES, BBB, and kidney infiltration. RES or macrophage system is made up of “blood monocytes, macrophages, and other immune cells” [160]. MPS in the liver, spleen, or lungs react with the drugs and activate “macrophages or leukocytes” that rapidly remove the drug. This leads to a short half-life of the drug [161]. To overcome this, NPs with “surface modification,” such as PEG, bypass this mechanism and increase the “drug half-life.” Besides, kidney infiltration is a crucial function in the human body. Proper kidney infiltration thus minimizes the toxicity caused by NPs.
The brain-blood barrier (BBB) is a specialized protection structure offered to protect the CNS from harmful and toxic agents. “Brain capillary endothelial cells” are arranged in the form of a wall that provides essential nutrients to the brain. Since the primary function of BBB is to block toxic agents to reach the brain, currently available chemotherapy agents for brain cancer are highly limited to intraventricular or intracerebral infusions [162]. However, NPs are known to cross BBB. Now, several approaches such as EPR effect, focused ultrasound, peptide-modified endocytosis, and transcytosis are used to deliver NPs. Glutathione PEGylated liposome encapsulated with methotrexate showed improved methotrexate uptake in rats [163]. Au-NPs are often used as they have proven to help transport drugs to induce apoptosis [164].
NPs being carriers also increase the drug stability by preventing the degradation of the encapsulated cargo. Additionally, a large volume of drugs can be encapsulated without any chemical reaction. Dry solid dosage forms are more stable than nanoliquid products [165]. Stabilizers can be used to enhance stability. Yet another way to increase stability is to use porous NPs.
Tumor has unique pathophysiology features such as extensive angiogenesis, flawed vascular architecture and defective lymphatic drainage. The NPs use these features to target tumor tissue. Due to reduced venous return in tumor tissue and meager lymphatic clearance, NPs are effectively retained. This phenomenon is known as EPR. Similarly, by targeting the adjacent tissues, tumor-targeting can be accomplished [166].
NPs can be administered through several routes like oral, nasal, parenteral, intra-ocular etc. NPs have a high surface-to-volume ratio and intracellular uptake. Studies have reported that NPs are more effective than microparticles as drug carriers [167].
Nanoparticles in Immunotherapy
The immune system sets an important part in the establishment and development of cancer cells. The advancement of immunotherapy has revolutionized cancer therapy. It is found that NPs not only help in target delivery of chemotherapy but can also be used in combination with immunotherapy. There are several approaches in immunotherapy aimed at activating the immune system against cancer cells [168] by “immune checkpoint blockade therapy,” “cancer vaccine therapy,” “chimeric antigen receptor (CAR)-T cell therapy,” and “immune system modulator therapy” [169,170,171]. NP-based immunotherapy includes “nanovaccines,” “aAPCs (artificial antigen-presenting cells),” and “immunosuppressed TME targeting.”
Nanovaccines specialize in delivering “tumor-associated antigens” and “adjuvants” to antigen-presenting cells, such as dendritic cells (DCs) [172]. Moreover, these can also be employed as adjuvants to enhance “APC antigen presentation” and promote DC maturation that leads to the stimulation of cytotoxic T cells that have anti-tumor function [173, 174]. Liposomes, PLGA NPs, gold NPs are found to have the ability to deliver TAAs into DCs in the cytoplasm [175]. Mesoporous silica, the most used inorganic NP, has exhibited an adjuvant role, leading to immune response stimulation [176]. Artificial APCs interact with MHC-antigen complexes directly which binds to T cells. They also bind to co-stimulatory molecules that bind to co-stimulatory receptors leading to T cell activation [177]. Targeting the immunosuppressed TME is yet another method of using NPs in immunotherapies. This is done by targeting essential cell types in TME such as “tumor-associated macrophages (TAMs),” regulatory T cells, and “myeloid-derived suppressor cells (MDSCs).”
Besides, the combination of chemoimmunotherapy has been demonstrated to be a capable approach in cancer therapy. For instance, a study has shown that co-loading Nutlin-3a, which is a chemotherapeutic agent and cytokine GM-CSF, in “spermine-modified acetylated dextran (AcDEX) NPs” improved cytotoxic CD8( +) T cells proliferation and activated an immune response [178].
“Programmed cell death protein 1 (PD-1)” and “programmed cell death ligand 1 (PD-L1)” are some of the essential immune checkpoints [179]. Hence immune checkpoint inhibitors are used to target these using NPs. According to a study, conventional immune checkpoint inhibitors of PD-L1/PD-1 displayed inconsistent responses. To enhance the chances and bonding of immune checkpoint inhibitors and immune checkpoints, multivalent poly (amidoamine) dendrimers were used. Usage of these dendrimers not only showed enhanced PD-L1 blockade but also showed improved drug accumulation at the tumor site [180].
Nanoparticles in Cryosurgery
Cryosurgery is an advanced practice of freeze-destroying cancer tissue. Although this is less invasive and causes intraoperative bleeding and postoperative complications, certain drawbacks like inadequate freezing capacity and damage to adjacent cells need to be addressed [181]. The rise of nanotechnology has enabled the use of NPs in cryosurgery.
The primary working of nanocryosurgery is introducing NPs with particular properties into the cancer cells and causing freezing [182]. During this process, ice is formed within the cells, which causes damage to it. This is an important process and can be carried out effectively using NPs. The thermal conductivity property of NPs can be exploited, which significantly freeze the tumor tissue and cause tumor damage [183]. Besides, they cool down rapidly, and it is feasible to regulate the “growth direction” and “direction of the ice ball” (Fig. 6).
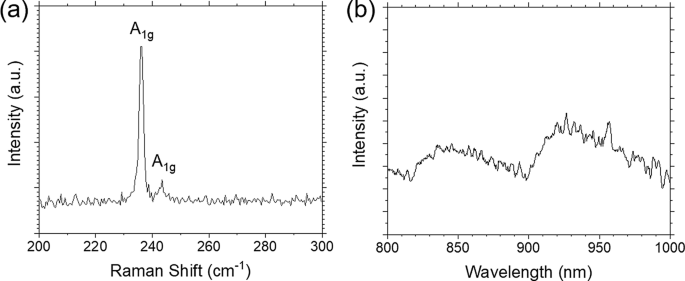
Diagrammatic representation of NPs in cryosurgery
When the location of the tumor makes it not feasible for cryosurgery or if other adjacent organs are at risk, there are high chances that the freezing can damage healthy tissue. Recently, phase change materials (PMs) made up of NPs are used to protect the adjacent normal healthy tissue during cryosurgery [184]. For instance, liposome-based microencapsulated phase change NPs have shown incredible results in protecting surrounding healthy tissue [185]. These NPs are deemed to possess large latent heat and low thermal conductivity, making them perfect for cryosurgery.
Significant Challenges in the Clinical Application of Nanoparticles
At present, as nanotechnology has bloomed, the amount of knowledge and research put into nanoparticles has steeply raised. But only a few of them actually make it up to clinical trials. Most of them only halt at in vivo and in vitro stages. Each individual nanoformulation has particular challenges in their clinical translation, but most NPs face similar challenges that can be divided into biological, technological, and study-design related.
Biological challenges include lack of routes of administration, tempering biodistribution, the channel of NPs across the biological barriers, their degradation, and toxicity [186]. NPs are usually injected via intravenous injections directly into the blood, which takes away NPs, making it challenging to stay and interact with the target site. As a result, a high concentration drug is used, which might not provide desired therapeutic effects [187]. However, magnetic NPs can be used to overcome this as many in vivo and in vitro studies have proved the usage of 3D magnetic fields to control the movement of NPs against blood flow. But, the effect of magnetic fields on the human body, crosstalk between magnetic fields, and a large number of NPs has to be researched upon.
Controlling the biological fate of NPs is very hard and needs a lot of focus. Even though NPs are made up of biosafety materials and are modulated accordingly to increase the retention time and half-life, there runs a risk of lung, liver and kidney damage. Some factors that govern toxicity are surface area, particle size and shape, solubility, and agglomeration [188]. NPs have shown greater deposition in the lung with inflammatory, oxidative and cytotoxic effects [189]. Studies reveal that healthy cells often suffer from free radicals generated by NPs [190]. Fabricating NPs with more biocompatible substances like chitosan and materials that disintegrate after near infrared light irradiation may be potential solutions.
Another tricky challenge is avoiding the “mononuclear phagocytic system (MPS).” In biological fluids, NPs adsorb proteins to produce PC, which attacks MPS to uptake NPs. To escape this, NPs have been coated with materials that prevent the formation of the protein corona. However, they have not shown any significant results. Designing NPs that target “macrophages” and using those as new drug vehicles can be pitched to overcome this problem. Currently, preventing macrophage recruitment, depleting and reprograming TAMs, and obstructing “CD47-SIRPα pathways” are commonly used strategies [191].
Technological challenges of NPs include scale-up synthesis, equal optimization, and performance predictions. These are very crucial in safeguarding the clinical success of NPs. Most of the NPs that are used in vivo and in vitro studies are usually produced in minor batches, and scale-up for huge quantities is not constantly feasible given instrumentation and other reasons. The lead clinical candidates that prove to be the best in animal models are not systematically designed optimized. To overcome this, we can use certain methods that can test numerous nanoformulation and by selective iterations selecting a single optimized formulation [192,193,194]. However, such hits shouldn’t be introduced directly in human testing. Predicting nanoparticle efficacy and performance is hard and replicating the in vivo results in human trials is a herculean task. Computational or theoretical modeling along with experimental results can be designed to imitate physiological tissue and surrounding. For instance, organs-on-chips are being actively studied and can improve NP predictions of efficacy and performance.
Study-design challenges like study size, intent, and timing of NP therapies during the therapy impact significantly during clinical studies. Most of the studies revolve around “cell and animal models” that may not provide comprehensible results in human trials. Therefore, the usage of a single model is tough to imitate natural reactions in the human body. In addition, “models of cancer metastasis” should be actively researched as metastasis is one of the significant properties of cancer. Moreover, N = 1 clinical studies will be required if we focus on personalized medicine. This needs to count in many factors such as genetic, environmental, and past medical history. [195, 196]. Another major challenge is that NPs are never used as first-line therapies. Although we have effectively approved nanoformulations, they are usually saved for further treatment if disease progression is found in the clinical trial scenario. Most of the patients have either had progressed on multiple lines of therapies or have gained drug resistance. These situations often skew the clinical trial results and lessen the chance of NP treatment to benefit those who are likely still treatable.
Conclusion and Future Perspective
Nanotechnology has shown a promising new era of cancer treatment by delivering small molecules for cancer detection, diagnosis, and therapy. Cancer therapies based on the exceptional features of NPs are being vastly used in the clinical setting of several cancer types. NP-based DDS is linked with enhanced pharmacokinetics, biocompatibility, tumor targeting, and stability compared to conventional drugs. Moreover, NPs provide an excellent platform for combination therapy which helps in overcoming MDR. With increasing research, several types of NPs, such as polymeric NPs, metallic NPs, and hybrid NPs, have shown improved efficacy of drug delivery. Researchers must be well attentive to the features of the nominated nanoplatforms and the properties of therapeutic agents. However, there are certain limitations like deficiency of in vitro models that precisely replicate in vivo stage, immunotoxicity, the long-term toxicity, and neurotoxicity. Although “nanovaccines” and “artificial APCs” have proved improved efficacy compared to conventional immunotherapy, the clinical efficacy is substandard. The safety and tolerance of these new modalities should to be inspected. Additionally, developing “immunomodulatory factor-loaded NPs” may advance the efficiency of vaccines for immunotherapy.
This is an emerging area, and it is anticipated that with growth in proteomics research on the “mechanism of cancer origin, MDR, occurrence,” more NP-based drugs can be exploited. Compared to the mammoth amount of investigations, only a few NP-based drugs are actually in use, a few others in clinical trials, and most in the exploratory stage. For rational nanotechnology design, more efforts must be reserved in “understanding toxicity, cellular and physiological factors that regulate NP-based drug delivery, EPR, and PC mechanism” in the human body. Based on the evidence cited above, we presuppose that the revolution in clinical translation for NP-based cancer therapy will be attained with nanotechnology and cancer therapy development.
Availability of Data and Materials
Not applicable.
Nanomaterialen
- De vijf belangrijkste problemen en uitdagingen voor 5G
- Demonstratie van een flexibele op grafeen gebaseerde biosensor voor gevoelige en snelle detectie van eierstokkankercellen
- Multifunctionele gouden nanodeeltjes voor verbeterde diagnostische en therapeutische toepassingen:een overzicht
- Vooruitgang en uitdagingen van fluorescerende nanomaterialen voor synthese en biomedische toepassingen
- Met resveratrol geladen albumine-nanodeeltjes met verlengde bloedcirculatie en verbeterde biocompatibiliteit voor zeer effectieve gerichte pancreastumortherapie
- Nieuwe biocompatibele Au Nanostars@PEG-nanodeeltjes voor in vivo CT-beeldvorming en eigenschappen voor nierklaring
- Synthese en in vitro prestaties van met polypyrrool gecoate ijzer-platina nanodeeltjes voor fotothermische therapie en foto-akoestische beeldvorming
- 5-aminolevulinezuur-squaleen nanoassemblages voor tumorfotodetectie en therapie:in vitro studies
- Invloed van Mg-doping op ZnO-nanodeeltjes voor verbeterde fotokatalytische evaluatie en antibacteriële analyse
- Voorbereiding en karakterisering van kikkervisjes- en bolvormige hemin-nanodeeltjes voor verbeterde oplosbaarheid
- De voordelen en uitdagingen voor hybride productie begrijpen