Een recensie over de elektrochemisch zelfgeorganiseerde Titania nanobuisarrays:synthese, modificaties en biomedische toepassingen
Abstract
Titania-nanobuisjes die door anodische oxidatie zijn gegroeid, hebben de materiaalwetenschappelijke gemeenschap geïntrigeerd door de vele unieke en potentiële eigenschappen, en de synthese van technologie versmelt tot zijn volwassen stadium. De huidige recensie zal zich richten op TiO2 nanobuisjes gegroeid door zelfgeorganiseerde elektrochemische anodisatie van Ti-metaalsubstraat, wat de synthese van dit type zelfgeorganiseerde titanium nanobuislagen en de middelen om de grootte, vorm, de mate van orde en gekristalliseerde fasen te beïnvloeden kritisch benadrukt door de anodisatie aan te passen parameters en de daaropvolgende thermische gloeiing. De relatie tussen afmetingen en eigenschappen van de anode TiO2 nanobuis-arrays zullen worden gepresenteerd. De laatste voortgang en betekenis van het onderzoek naar het vormingsmechanisme van anodisch TiO2 nanobuisjes worden kort besproken. Daarnaast zullen we de meest veelbelovende toepassingen laten zien die recentelijk in biomedische richtingen zijn gerapporteerd en modificaties die zijn uitgevoerd door doping, oppervlaktemodificatie en thermisch uitgloeien om de eigenschappen van anodisch gevormd TiO2 te verbeteren. nanobuisjes. Eindelijk worden enkele onopgeloste problemen en mogelijke toekomstige richtingen van dit veld aangegeven.
Inleiding
Sinds het begin van de twintigste eeuw is titaandioxide (TiO2 ) is gebruikt als commerciële productie in zonneblokkers, verven, sensoren, fotokatalyse, zonnecellen, elektrochrome apparaten, medicijnafgifte, enz. [1,2,3,4,5,6,7]. Het fenomeen dat TiO2 kan de fotogegenereerde elektron-gatparen produceren onder bestraling met licht, kan helpen water te splitsen in zuurstof en waterstof, waardoor de energiecrisis in de toekomst als de meest potentiële brandstof kan worden opgelost. Fujishima en zijn collega's rapporteerden voor het eerst de fotokatalytische watersplitsing op een TiO2 elektrode onder ultraviolet (UV) licht [8,9,10], en sindsdien is titaniumdioxide een van de meest bestudeerde verbindingen in de materiaalkunde geworden. Van alle overgangsmetaaloxiden vertoont het een breed scala aan functionele eigenschappen zoals chemische inertie, corrosieweerstand en stabiliteit, met name de verbetering van biocompatibiliteit [11], en elektrische en optische eigenschappen [1]. Sinds Iijima in 1991 koolstofnanobuisjes [12] ontdekte, die een unieke combinatie vertonen tussen vorm en functionaliteit, waarbij eigenschappen direct door de geometrie kunnen worden beïnvloed, zijn er enorme inspanningen geleverd op het gebied van nanotechnologie, voornamelijk in de chemische, fysische en biomedische materiaalwetenschap.
Hoewel het meest onderzochte nanomateriaal tot nu toe koolstof is, heeft een andere klasse van nanobuisvormige materialen, die meestal gebaseerd zijn op overgangsmetaaloxiden, de afgelopen 20 jaar aanzienlijke belangstelling gewekt. De eerste poging om geanodiseerde titanium nanobuisjes te vormen werd gedaan door Assefpour-Dezfuly [13] die een alkalische peroxidebehandeling gebruikte, gevolgd door elektrochemische anodisatie in een elektrolyt dat chroomzuur bevat. En aangezien Zwilling et al. rapporteerde dat ze in 1999 de eerste zelf-georganiseerde nanobuislagen op Ti-substraat produceerden door elektrochemische anodisatie in chroomzuurelektrolyten die fluorionen bevatten, is het veld enorm snel uitgebreid [14]. In de afgelopen tien jaar zijn er meer dan 33.800 artikelen gepubliceerd met het trefwoord 'titania nanobuisjes'. Figuur 1 geeft de totale publicatie per jaar uitgesplitst op het gebied van TiO2 nanobuisjes en maakt een vergelijking tussen verschillende synthetische methoden in de periode 2002-2017, die niet alleen een exponentiële groeitrend laat zien, maar blijkbaar aangeeft dat de zelfgeorganiseerde anodische TiO2 nanobuisarrays krijgen veel aandacht met een groot potentieel en voordelen. De laatste tijd hebben Lee et al. heeft een uitgebreid en up-to-date beeld gegeven op het gebied van anodische titanium nanobuisjes die bijna alle aspecten omvatten, inclusief groei, modificaties, eigenschappen en toepassingen met een korte beschrijving van verschillende synthesebenaderingen [15]. Vergeleken met andere bereidingsmethoden zoals hydro/solvothermal [16,17,18] en template-assisted methoden [19, 20], blijkt directe oxidatie een eenvoudige techniek met een sterke operabiliteit op welke manier de gewenste controleerbare nanostructuur via het aanpassen van de grootte, vorm, en de mate van ordening kan worden vergroot door middel van optimalisatie van de oxidatieparameters zoals de aangelegde potentiaal, tijd, temperatuur, pH en de samenstelling van de elektrolyt [15]. Vanwege de specifieke geometrie vertegenwoordigen de zelf-uitgelijnde oxide-nanobuislagen met een zeer georganiseerde structuur en oppervlakte-volumeverhouding unieke eigenschappen, zoals een zeer hoge mechanische sterkte en het grote specifieke oppervlak, en bieden zelfs elektronische eigenschappen zoals hoge elektronen mobiliteitssnelheid of kwantumbeperkingseffecten [15, 21]. Bovendien is elektrochemische anodisatie een goedkoop proces en niet beperkt tot titanium, maar kan ook geschikt zijn voor andere overgangsmetalen Hf [22], Zr [23], Nb [24], Ta [25], V [26] of legeringen TiAl [27] en TiZr [28]. De huidige recensie zal nog steeds gericht zijn op TiO2 nanobuisjes gegroeid door zelfgeorganiseerde elektrochemische anodisatie van Ti-metaalsubstraat. Daarnaast zullen we de nadruk leggen op de synthese van dit type zelfgeorganiseerde titania nanobuislagen en de middelen om de grootte, vorm, de mate van orde en gekristalliseerde fasen te beïnvloeden via het aanpassen van de anodisatieparameters en de daaropvolgende thermische uitgloeiing, inclusief vier verschillende generaties verschillend van elektrolytensoorten en de gedefinieerde anodisatie in twee stappen, enz. De relatie tussen afmetingen en eigenschappen van het anodische TiO2 nanobuis-arrays zullen worden gepresenteerd. De laatste voortgang en betekenis van het onderzoek naar het vormingsmechanisme van anodisch TiO2 nanobuisjes worden kort besproken. We zullen de meest veelbelovende toepassingen laten zien die recentelijk zijn gerapporteerd in biomedische richtingen en modificaties die zijn uitgevoerd door doping, oppervlaktemodificatie en thermisch uitgloeien om de eigenschappen van anodisch gevormd TiO2 te verbeteren. nanobuisjes. We houden ook rekening met onopgeloste problemen en mogelijke toekomstige richtingen op dit gebied. De tekst van de hoofdparagraaf volgt hier direct.
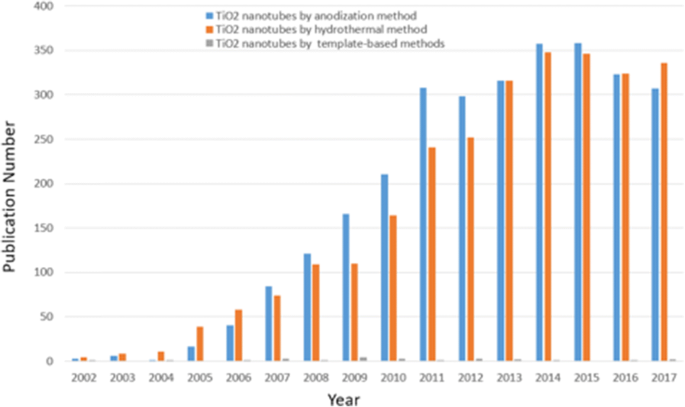
Onderzoekstrend. Het aantal papieren uitgesplitst per jaar gerelateerd aan TiO2 nanobuisjes gedifferentieerd door verschillende synthesemethoden van 2002 tot 2017. (Gegevens werden verzameld uit Science Citation Index Expanded met behulp van titanium nanobuisjes en anodisatie- of hydrothermische methoden of op sjabloon gebaseerde methoden als trefwoorden)
Synthese van TiO2 Nanobuis-arrays door elektrochemische anodisatie
In de afgelopen jaren, terwijl vele verschillende vormen van nanogestructureerde titaandioxide waaronder nanostaafjes, nanodeeltjes, nanodraden en nanobuisjes met succes zijn ontwikkeld [29,30,31], hebben nanobuisjes steeds meer belangstelling gekregen voor technologische toepassingen dankzij de unieke zelf-geassembleerde structuur met een groot grensvlak en gemakkelijke controle van de grootte en vorm, die als een betere kandidaat kunnen worden toegepast op oppervlakteafhankelijke toepassingen. Er zijn een aantal uitstekende recensies [1, 2, 15, 32,33,34] beschikbaar voor het omgaan met de functies van TiO2 nanomaterialen gecategoriseerd met verschillende synthetische methoden. Het is bewezen dat elektrochemische anodisatie een van de meest effectieve methoden is om de titanium nanobuisjes te verkrijgen als een relatief eenvoudige technologie die gemakkelijk kan worden geautomatiseerd. We zullen de belangrijkste technieken specificeren om anodische TiO2 . te fabriceren nanobuisjes hieronder.
Zelfgeorganiseerde anodische TiO2 Nanobuis-arrays
Zoals uitgebreid bestudeerd, kunnen de titanium nanobuislagen worden gevormd onder een specifieke set omgevingsomstandigheden. Het oxidatieapparaat bestaat uit drie delen:(I) een systeem met drie elektroden met de geprepareerde Ti-folie als de werkelektrode die wordt ontvet door achtereenvolgens te soniceren in aceton, ethanol en gedeïoniseerd water, platina als de tegenelektrode en gewoonlijk Ag/AgCl als referentie-elektrode (Fig. 2a), terwijl de pH-elektrode soms wordt toegevoegd om de uiteindelijke concentratie van F − te verkrijgen en HF [35] of een ander eenvoudig systeem met twee elektroden bestaande uit Ti-folie als anode en inerte metaalelektrode als kathode (Fig. 2b) [36]; (II) in het algemeen fluoride-ionen, chloride-ionen, chroomionen, bromide-ionen of perchloraat bevattende elektrolyten; en (III) een gelijkstroomvoeding. Er zijn twee hoofdkenmerken die worden beïnvloed door de anodisatiecondities van vorming die de veelbelovende toepassingen van titanium nanobuisjes beïnvloeden:(I) geometrie:grootte, vorm, de mate van orde, gekristalliseerde fasen, enz. en (II) eigenschappen in chemische, fysische en biomedisch. Met andere woorden, via het regelen van de elektrochemische anodisatieparameters (toegepaste potentiaal, de duur van anodisatie, elektrolytsysteem inclusief de concentratie van de fluorionen en water in de elektrolyt, elektrolyttemperatuur, elektrolyt-pH, enz. Die in meer detail zullen worden besproken in de sectie "Synthese van TiO2-nanobuisarrays door elektrochemische anodisatie", kan men verschillende titanium nanostructuren fabriceren, zoals een plat compact oxide [1], een poreuze laag [1, 36], ongeordend TiO2 nanobuislagen die in bundels groeien [37], of eindelijk een goed georganiseerde regelmatige TiO2 nanobuisjes of geavanceerde nanobuisjeslaag:vertakte buis [38], bamboe-achtige [38, 39], dubbelwandige [40], nanolace [38], of dubbellaagse [39] structuren waarmee eigenschappen anders gevonden kunnen worden. Figuren 3 en 4 tonen veldemissie scanning elektronenmicroscopie (FE-SEM) beelden van de typische voorbeelden van dergelijke TiO2 morfologieën van nanobuisjes.
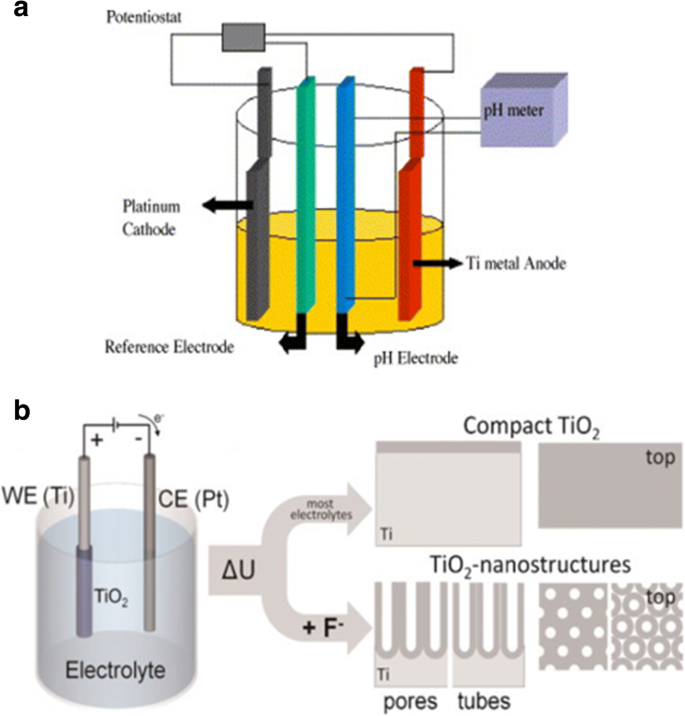
Schematisch opgezet. een Illustratieve tekening van een systeem met drie elektroden met de geprepareerde Ti-folie als werkelektroden, platina als tegenelektrode en meestal Ag/AgCl als referentie-elektrode, terwijl de pH-elektrode als pH-meter. Overgenomen van ref. [35]. b Illustratieve tekening van een eenvoudig twee-elektrodensysteem bestaande uit Ti-folie als anode en inerte metaalelektrode als kathode. Anodisatie leidt tot verschillende geanodiseerde oxidelagen onder verschillende omstandigheden. In de meeste neutrale en zure elektrolyten kan een compacte titania worden gevormd. Maar als verdunde fluoride-elektrolyten worden gebruikt, zullen nanotubulaire / nanoporeuze oxidelagen direct aan het metalen oppervlak worden gehecht. Overgenomen van ref. [36]
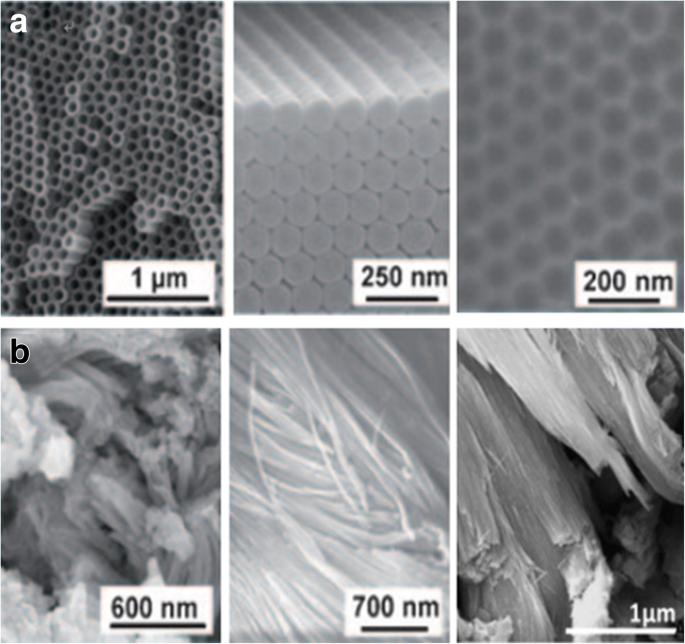
SEM-afbeeldingen van geanodiseerde TiO2 nanobuislagen door verschillende anodisatieprocessen van Ti. een De zeer geordende TiO2 nanobuisjes (in boven- en zijaanzicht) worden verkregen in organische elektrolytsystemen, met zelfgeordende oppervlaktekuiltjes (rechts) die in feite metalen oppervlakken zijn wanneer de buislagen worden verwijderd. Overgenomen van ref. [1]. b De ongeordende TiO2 nanobuisjes worden in patches op het oppervlak gekweekt en samengesmolten tot bundels in chloride-bevattende elektrolyt door een ultrasnelle anodisatietechniek die bekend staat als rapid-breakdown anodization (RBA). Overgenomen van ref. [1] en [37]
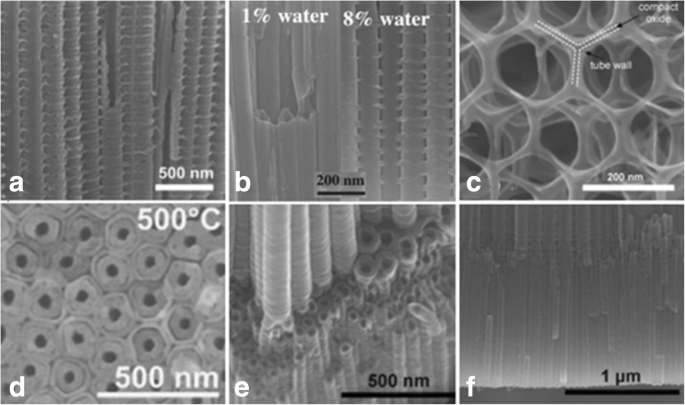
SEM-afbeeldingen van geavanceerde TiO2 morfologieën van nanobuisjes. een Met bamboe versterkt TiO2 nanobuisjes zijn vervaardigd onder specifieke wisselspanning (AV) omstandigheden in ethyleenglycol bestaande uit 0,2 mol/L HF, met een opeenvolging van 1 min bij 120 V en 5 min bij 40 V. Overgenomen van ref. [38]. b Overgang van gladde naar bamboe-achtige TiO2 nanobuisjes kunnen worden geïnduceerd door anodisatie met gecontroleerde watertoevoeging (watergehalte:1 tot 8%) tot een 0,135 M NH4 F/ethyleenglycol elektrolyt
overgenomen van ref. [39]. c De 2D nanolace-structuren worden verkregen onder spanningscycli die gedurende een langere periode worden uitgevoerd in de fluoridehoudende elektrolyt, met een reeks van 50 s bij 120 V en 600 s bij 0 V. Overgenomen van ref. [38]. d De dubbelwandige TiO2 nanobuisjes worden gekweekt door anodisatie van Ti in een fluoride bevattende ethyleenglycolelektrolyt bij 120 V na uitgloeien bij 500 °C met een verwarmingssnelheid van 1 °C s −1 . Overgenomen van ref. [40]. e De vertakte nanobuisjes kunnen worden waargenomen door spanningsstappen, eerst bij 120 V (6 uur) en vervolgens bij 40 V (2 uur). Overgenomen van ref. [38]. v De dubbellaagse nanobuisjes met gelijke of twee verschillende buisdiameters zijn te zien. Overgenomen van ref. [38]
(Momenteel is TiO2 nanobuisarrays met buisdiameters variërend van 10 tot 500 nm, dikte van lagen variërend van enkele honderden nanometers tot 1000 m en wanddikte variërend van 2 tot 80 nm kunnen worden verkregen [15, 41].)
Het was twee decennia geleden toen Masuda en Fukuda voor het eerst melding maakten van het zeer geordende poreuze aluminiumoxide door de anodisatie-omstandigheden optimaal aan te passen [42]. Later hebben onderzoekers hun best gedaan om ook voor TiO2 . vergelijkbare structuren te maken nanobuis lagen. En er zijn drie cruciale factoren die de mate van orde in anodische TiO2 . beïnvloeden nanobuisarrays (in overeenstemming met polygonen in de lagen en de standaarddeviatie in buisdiameter):het Ti-substraat, de aangelegde spanning en de herhaalde anodisatie [33, 43]. Het is duidelijk dat er minder fouten in de opstelling kunnen worden verkregen voor materiaal met een hoge zuiverheid bij de hoogst mogelijke spanning onder diëlektrische doorslag [33] en de idealiter zeshoekige zelfgeordende TiO2 nanobuisjes zoals getoond in Fig. 5 kunnen aanzienlijk worden verbeterd door secundaire buisgroei [43]. Sopha et al. toonden aan dat onzuiverheden de resulterende verschillende afmetingen en volgorde van nanobuisjes sterk beïnvloeden na de tweede anodisatie [44]. Bovendien is gebleken dat de kristallografische oriëntaties van de Ti-substraatkorrels cruciale effecten zijn op de groeikenmerken van TiO2 nanobuisarrays door elektronenterugverstrooiingsdiffractie (EBSD). Leonardi et al. ontdekte dat nanobuisjes alleen kunnen worden waargenomen met een oriëntatie die het mogelijk maakt een klepmetaaloxide te vormen op korrels waardoor fluoride-ionen kunnen doordringen door de oxidatiefilm waar 1 M (NH4 )H2 PO3 +0,5 gew.% NH4 F werden gebruikt als elektrolyt [45]. Evenzo rapporteerden Macak en collega's dat de groei van nanobuisjes op korrels niet wordt vertraagd in de veelgebruikte elektrolyt op basis van ethyleenglycol in vergelijking met het gebruik van waterige elektrolyt, zoals bekend uit de laatste literatuur [46]. Op de gepolijste Ti-plaat bleken korrels met [0 0 0 1]-oriëntatie of dichtbij deze de ideale korrels te zijn en het gebruik van monokristallijn Ti met ideale oriëntatie zou een grote vooruitgang zijn om de meest uniforme nanobuisarrays te verkrijgen [46] .
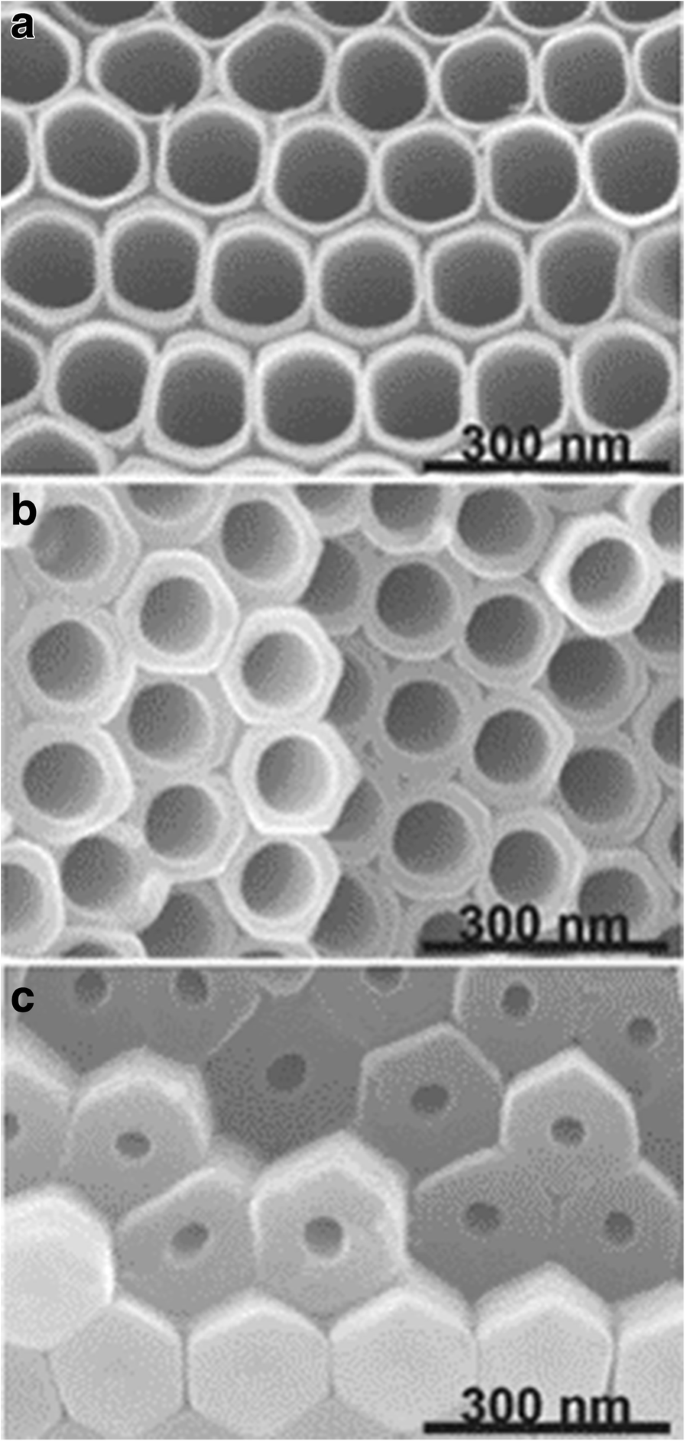
SEM-afbeeldingen van TiO2 nanobuisjes. De nanobuisjes zijn gevormd in ethyleenglycolelektrolyten die 0,27 M NH4 . bevatten F door herhaalde anodisatie van Ti. De doorsneden zijn genomen aan de bovenkant van de laag, in het midden en aan de onderkant van de laag. Overgenomen van ref. [43]
Niettemin zijn er nog enkele gebreken die de mate van orde beïnvloeden. De laatste tijd is het verder uitgebreid door uniforme nano-imprinting Ti. Kondo et al. bedacht een verwerkingscapaciteit van een ideaal geordende anode TiO2 door nano-imprinting van het Ti-oppervlak of een tweelaags exemplaar met een Al-laag aan de bovenkant en de Ti-laag aan de onderkant met behulp van een Ni-mal met geordende convexe. En de TiO2 lagen zouden op een meer geordende manier kunnen worden gegenereerd, waarbij de ondiepe concaven van het voorgestructureerde patroon als initiatieplaatsen fungeerden door de daaropvolgende anodisatie in NH4 F ethyleenglycoloplossing [47, 48]. Op de voet volgen Sopha et al. bedekt eerst een TiN-beschermende laag op Ti-substraat bereid door atomaire laagafzetting (ALD) vóór de pretexturering uitgevoerd door gefocusseerde ionenstraal (FIB) en de daaropvolgende anodisatie met behulp van ethyleenglycolelektrolyt om perfect hexagonaal gerangschikte nanobuislagen met een dikte te produceren van 2 m, wat de nanobuisjes kan beperken om alleen op de gegeven initiatieplaatsen te groeien en de anodisatietijd zonder defecten te verlengen [49].
Vormingsmechanisme van anodische TiO2 Nanobuisjes
Anodische oxidatietechnologie en onderzoek naar het vormingsmechanisme van anodische TiO2 nanobuisjes hebben lange tijd brede aandacht gekregen vanuit een grote diversiteit aan disciplines. Het mechanisme-onderzoek dat Diggle in 1969 rapporteerde over de films van compact anodische oxide en poreus anodische oxide [50] speelt nu nog steeds een uiterst belangrijke leidende rol. Uit een aanzienlijk aantal recente werken blijkt dat de overgang van poriën naar buizen geleidelijk verloopt [1, 27, 36]; het volledig theoretische model en de redenering werden echter niet gegeven.
Conventionele field-assisted dissolutie (FAD) is de meest acceptabele theorie [1, 33, 51]. Dat is in het proces van elektrochemische anodisatie, TiO2 nanobuisarrays worden gevormd door zelforganisatie van titania vanwege drie relatief onafhankelijke procedures:elektrochemische oxidatie van Ti in TiO2 , de door het elektrisch veld geïnduceerde oplossing van TiO2 , en de door fluorionen geïnduceerde chemische oplossing van TiO2 , het bereiken van een delicaat evenwicht. Als een karakteristieke stroomtijdcurve getoond in Fig. 6 voor elektrolyten die fluoride bevatten die leiden tot nanobuisvorming [51] en een typisch beeld dat kan helpen om het vormingsproces schematisch te illustreren [33] getoond in Fig. 7, kan de transiënt worden verdeeld in drie verschillende fasen:(I) In het eerste deel is er een stroomverval, veroorzaakt door een nieuw gevormd barrière-oxide, wanneer de twee belangrijkste processen, de binnenwaartse migratie van O 2− ionen naar het metaal/oxide grensvlak en de buitenwaartse migratie van Ti 4+ ionen naar het oxide/elektrolyt-grensvlak, een evenwicht bereiken. (II) In het tweede deel begint de stroom weer te stijgen met een vertraging veroorzaakt door het toenemende oppervlak van de anode. Hoe korter de vertraging is, hoe hoger de fluorideconcentratie zal zijn vanwege de door fluoride geïnduceerde oplossing van het gevormde TiO2 en poriën beginnen willekeurig te fabriceren, wat vervolgens de initiële vorming van TiO2 blijkt te zijn nanobuisjes. (III) Dan bereikt de stroom een stabiele toestand, wanneer de poriegroeisnelheid aan het metaaloxide-grensvlak en de geïnduceerde oplossnelheid van het gevormde TiO2 aan het buitenste grensvlak een evenwichtssituatie bereiken. De uiteindelijke buizen worden dus in toenemende mate v-vormig, dat wil zeggen dat de toppen van de buizen aanzienlijk dunnere wanden hebben dan hun bodems waar de buizen gesloten verpakt zijn. De gradiënt in de buiswanddikte in Fig. 5 kan worden toegeschreven aan verschillende blootstellingstijd en concentratie aan de elektrolyten langs de buizen [43].
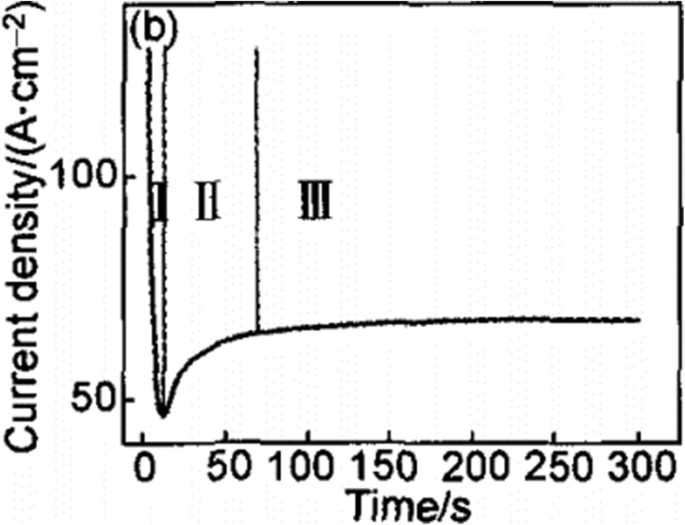
Typische stroomtijdcurve onder een constante spanning in elektrolyten die fluoride bevatten. De transiënt kan worden onderverdeeld in drie verschillende regio's (I –III ). (Ik ) In het eerste deel is er een scherp stroomverval. (II ) In het tweede deel begint de stroom met enige vertraging weer te stijgen. (III ) In het derde deel bereikt de stroom een stabiele toestand die wordt gereproduceerd uit ref. [51]
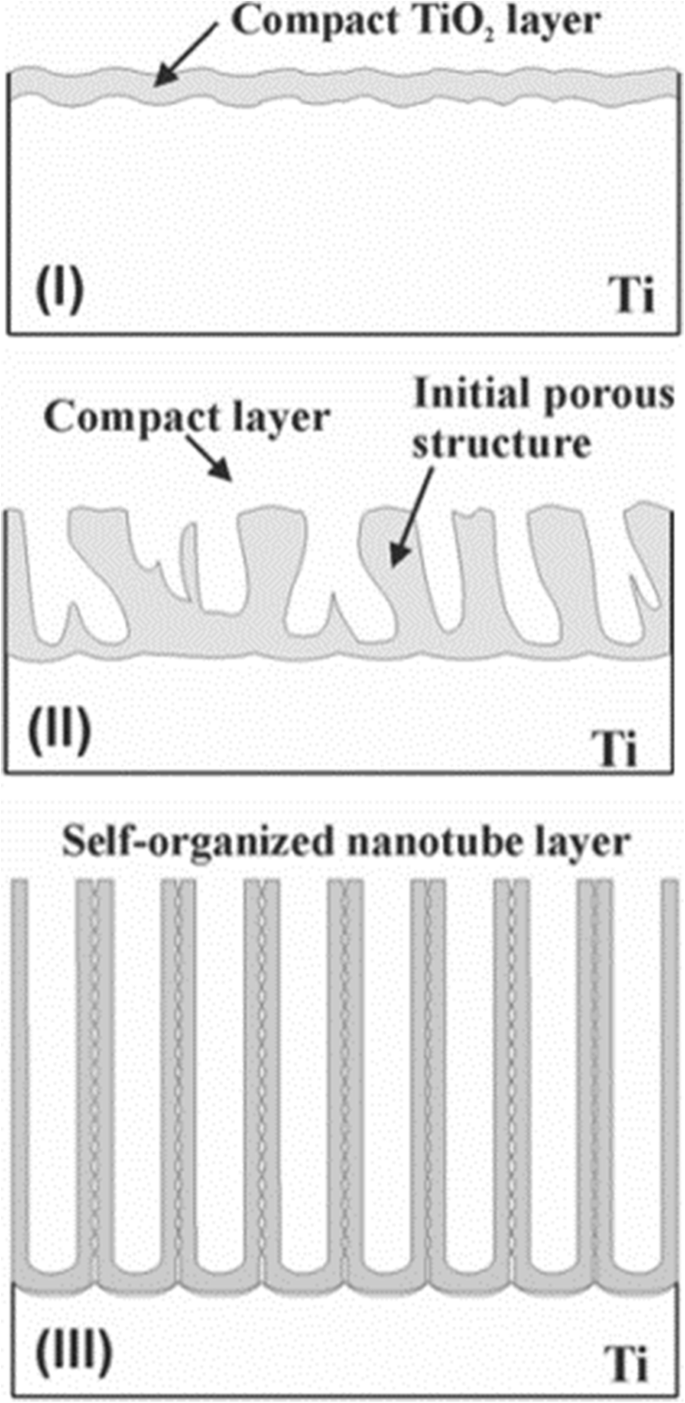
Het vormingsproces van TiO2 arrays van nanobuisjes. De vorming van TiO2 nanobuisarrays kunnen worden onderverdeeld in drie verschillende morfologische stadia (I –III ). (Ik ) Er wordt een barrièreoxide gevormd. (II ) Het oppervlak wordt lokaal geactiveerd en poriën beginnen willekeurig te groeien. (III ) Zelfgeorganiseerde nanobuislaag wordt gevormd gereproduceerd uit ref. [33]
Deze theorie kan het fenomeen van scheiding in buizen echter nog niet duidelijk verklaren, in tegenstelling tot een nanoporeuze structuur, en Fahim et al. merkte op dat het onder geschikte spanningen mogelijk is om titanium nanobuisjes te verkrijgen in zwavelzuuroplossing zonder fluoride-ionen, in welk geval de I-t-curve leek op degene die we zojuist hierboven hebben besproken [52]. Zoals Houser en Hebert opmerkten, zijn er nog geen groeimechanismen ontwikkeld om de kwantitatieve relaties tussen het proces van het titania-poreuze membraan en de I-t-curve te verklaren [53]. Omdat de interpretatie niet overtuigend genoeg is, verschijnen recentelijk nieuwe punten over het mechanisme zoals viskeuze stromingsmodel en groeimodel van twee stromen. Met betrekking tot deze mechanismen toont een recensie [51] veel beperkingen voor de traditionele veldondersteunde oplossingstheorie en geeft enkele verklaringen over de laatste voortgang en betekenis van het onderzoek naar het viskeuze stromingsmodel en het groeimodel van twee stromen.
Het effect van anodisatiecondities die geometrie en eigenschappen beïnvloeden
De samenstelling en concentratie van de elektrolyten hebben een significante invloed op de vorming van nanobuisjes. Afhankelijk van het verschil in elektrolyten dat we gebruiken, is de ontwikkeling in principe verdeeld in drie fasen:Tabel 1 geeft een samenvatting van de anodisatiecondities en afmetingen van het resulterende TiO2 nanobuisarrays in de drie generaties die tot nu toe door verschillende onderzoeksgroepen zijn onderzocht.
De eerste generatie:waterige elektrolyten op basis van fluorwaterstofzuur (HF)
De mijlpaal is dat Gong et al. presenteerde voor het eerst de uniforme titania-nanobuisarrays door anodische oxidatie van Ti in op HF gebaseerde waterige elektrolyten [54]. In HF-elektrolyten in waterige oplossingen, waar de pH relatief laag is, wat een hoge concentratie waterstofionen betekent, is de chemische oplossing van TiO2 geïnduceerd door fluorionen speelt de dominante status in het anodisatieproces [55]. Er werd in korte tijd een dynamisch evenwicht bereikt in het proces van het vormen van titanium nanobuisjes, en daarom werd de maximaal haalbare lengte van de nanobuisjes beperkt tot ongeveer 0,5 m [54.55.56].
De tweede generatie:gebufferde elektrolyten
In het daaropvolgende werk, om het chemisch oplossen van de buizen te verminderen, hebben Cai et al. toonde aan dat door het toevoegen van zwakkere zuren zoals KF of NaF aan een gebufferde oplossing en het aanpassen van de pH tot zwak zuur (pH =4,5) met zwavelzuur of natriumhydroxide, nanobuisjes van ongeveer 4,4 m lang werden bereikt [57]. De PH-waarde beïnvloedt de hydrolyse van de titaniumionen die het elektrochemisch etsen en chemisch oplossen blijken te verstoren. Cai et al. wees er ook op dat lagere pH-waarden kortere maar schone nanobuisjes produceren en hogere pH-waarden resulteren in langere nanobuisjes maar ongewenst afval [57]. Naarmate de pH-waarde stijgt, zal de hydrolysesnelheid toenemen, wat op zijn beurt de chemische oplossing vertraagt, wat leidt tot langere nanobuisjes, terwijl alkalische oplossing niet geschikt is voor de groei van nanobuisjes [57, 58]. Macak et al. hebben aangetoond dat in neutrale NaF-elektrolyt bij de juiste spanning veel langere nanobuisjes kunnen worden verkregen dan in zure oplossingen. [58]. Gegeven een bepaalde spanning in fluoridehoudende elektrolyt, kunnen door aanpassing van de pH-gradiënt de vereiste aspectverhoudingen en dikte van lagen worden bereikt [59].
De derde generatie:polaire organische elektrolyten
Elektrolyten zoals glycerol [59], dimethylsulfoxide [60], formamide of diethyleenglycol [61, 62], ethyleenglycol [41, 63], die fluorideverbindingen bevatten zoals NH4 F, NaF en KF verschijnen geleidelijk. Macak en collega's namen het voortouw bij het gebruik van viskeuze glycerolelektrolyt om titania-nanobuisarrays te fabriceren met een dikte van ongeveer 7 m en een gemiddelde buisdiameter van 40 nm [59]. Het is aangetoond dat een hogere aspectverhouding TiO2 nanobuisjes kunnen in zo'n polaire organische elektrolyt worden gekweekt vanwege een goede regeling van de pH van de elektrolyt, waardoor de chemische oplossing van het titania [64] wordt verminderd. Paulose et al. gevormde nanobuisjes van ongeveer 134 m lang, bereid met ethyleenglycol dat 0,25 gew.% NH4 bevat F bij een anodisatiepotentiaal van 60 V gedurende 17 uur [60]. Kort daarna, meer dan 250 μm dik TiO2 nanobuis-arrays werden gerapporteerd door Albu [65]. Bovendien speelt het watergehalte een dubbele rol in het proces:het is onmisbaar voor de vorming van titania, maar het versnelt ook het chemisch oplossen [63]. Daarom is het nodig om het effect van het watergehalte tot een minimale significantie te verkleinen om de dikte en mate van orde van de TiO2 arrays van nanobuisjes. Over het algemeen is het beperken van het watergehalte tot minder dan 5% de sleutel om met succes zeer lange nanobuisjes te bereiken [60], en een minimale hoeveelheid water (0,18 gew.%) is vereist om goed georganiseerde titanium nanobuisjes te vormen [66]. Er werd gemeld dat met de toevoeging van water de geregistreerde stroomdichtheid afnam, wat het hoogst was in watervrije ethyleenglycoloplossing [66]. Paulose et al. eerste gerapporteerde vorming van zelfgeorganiseerde hexagonale titania-nanobuisarrays met een lengte van ongeveer 1000 m bij 60 V gedurende 216 uur in ethyleenglycol met 0,6 gew.% NH4 F en 3,5% water [41]. Een ander opvallend fenomeen is dat gladde buiswanden groeien bij een laag watergehalte, terwijl rimpels op zijwanden worden gevormd bij een hoger gehalte, zoals weergegeven in Fig. 4b [59, 67]. Als verreweg het meest gebruikte type elektrolyten, leidt ethyleenglycol dat water en fluoride-ionen bevat altijd tot dubbelwandige nanobuisstructuren (Fig. 4d) [40, 68,69,70], terwijl de binnenste laag kan worden verwijderd door een geschikte gloeibehandeling gevolgd door een eenvoudig chemisch etsproces. Na verwijdering van de binnenschaal maken de verbrede buizen een laag-voor-laag decoratie met nanodeeltjes mogelijk met behulp van een repetitieve benadering op basis van TiCl4 -hydrolyse [71]. Terwijl enkelwandige buizen aanzienlijk verbeterde geleidbaarheid en elektronentransporttijden vertoonden in kleurstofgevoelige zonnecellen (DSSC's) [71, 72] waar de dikte van de hele buis in principe hetzelfde is en de binnenschaal niet langer verschijnt, Mirabolghasemi et al. maakte een vergelijking tussen de dubbel- en enkelwandige buizen en presenteerde gewenste enkelwandige buizen met toevoeging van dimethylsulfoxide (DMSO) aan elektrolyten met 1,5 M H2 O en 0,1 M NH4 F [72].
Onlangs is gemeld dat niet-fluoride-gebaseerde elektrolyten TiO2 . doen groeien nanobuisarrays die kunnen worden beschouwd als de vierde synthesegeneratie, waaronder zoutzuur, waterstofperoxide, perchloorzuuroplossingen en hun mengsels [73, 74]. Allama en Grimes beschreven goed ontwikkelde nanobuisarrays met een lengte van 300 nm, een binnendiameter van 15 nm en een buitendiameter van 25 nm die werden verkregen in een 3 M zoutzuur (HCl) waterige elektrolyt bij oxidatiespanningen tussen 10 en 13 V. Maar toevoeging van een lage concentratie H3 PO4 resulteerde in een verandering van nanobuisjes naar staafjes. Ze suggereerden verder dat ze niet in staat waren om zelfgeorganiseerde nanobuisarrays in HCl-bevattende elektrolyten te bereiken met een concentratie van lager of hoger dan 3 M [73]. Allama ontdekte dat het toevoegen van waterstofperoxide aan de zoutzuurbevattende waterige oplossing een mogelijke methode zou kunnen zijn om de titanium nanobuisjes te verlengen, die een sterk oxiderende eigenschap hebben na een dikkere oxidelaag, wat aantoont dat fluoride-ionen met succes kunnen worden vervangen door chloride-ionen in de groei van nanobuis-arrays [74]. Bovendien zijn ionische vloeistoffen zonder toevoeging van vrije fluoridesoorten de afgelopen jaren behandeld als een ander type oplosmiddelsysteem voor titanium nanobuisjes [75, 76].
Naast de standaardparameters is de geometrie van de resulterende nanobuisjes afhankelijk van het herhaalde gebruik van elektrolyt (het "gebruikte oplossingseffect"). In vergelijking met de buizen verkregen met verse oplossingen, met gebruikmaking van eenmaal gebruikte oplossingen, vertoonden ze een toename van de lengte van de nanobuisjes en een betere kwaliteit, waarbij de bereikte groeisnelheid van de nanobuisjes consistent hoger is voor eenmaal gebruikte oplossingen bij 60 V en hoger [77] en een iets ander maar te onderscheiden huidig voorbijgaand gedrag kon worden opgemerkt [66]. Bovendien werd in de tweemaal gebruikte oplossing geen nanotubulaire structuur maar een oxidefilm verkregen vanwege de uitputting van F − soorten [78]. Echter, Sopha et al. onderzocht verschillende leeftijden van op ethyleenglycol gebaseerde elektrolyten op de morfologie van TiO2 nanobuisjes die aantonen dat in oudere elektrolyten de arrays lagere aspectverhoudingen vertonen [79].
Toegepast potentieel
Anodisatiespanning is de kritische factor die de buisdiameters regelt [80, 81]. De afmeting van de nanobuis-arrays kan eenvoudig worden voorspeld door het geschikte spanningsbereik genaamd potentiaalvenster over de elektrode toe te passen [67]. Bij een lage spanning vindt minder ontbinding van het elektrische veld plaats, waardoor TiO2 . wordt gevormd nanobuisjes met kleinere diameters. Als de spanning te laag is, kan de TiO2 laag wordt compact maar er kan geen nanotubulaire structuur worden waargenomen. Integendeel, een sponsachtige poreuze structuur zal worden gezien wanneer de spanning te hoog is. Bij regelbare spanning is de diameter van de nanobuisjes evenredig met de spanning [81]. Furthermore, studies show that the range of voltage forming nanotubes is also related to the electrolyte system. In aqueous electrolytes, the potential window should be controlled from 10 to 25 V, which in organic electrolytes is much wider between several volts and some hundred volts. Wang and Lin found out the fact that in aqueous electrolytes, the anodization potential exhibits significant influence on the growth of TiO2 nanotube arrays, which exhibited slight influence in non-aqueous electrolytes in this regard [82]. The voltage dependence has a significant reduction in non-aqueous electrolytes which is attributed to a large extent to the low conductivity of organic electrolytes [83, 84].
The Duration on Anodization
The duration of anodization affects the nanotubes mainly in two aspects:(I) the formation of the tubes or not and (II) the length of the tubes. That is, in the early stage of the anodization, a compact TiO2 film is formed. If the duration is too short to reach an equilibrium in reaction, the regular nanotube array cannot be achieved instead of a disordered porous layer [67]. With increasing the anodization time, porous structure gradually grows deeper and converts into the TiO2 nanotubular array [1, 33, 51]. If other electrochemical parameters are kept unchanged, increase in the nanotube length is observed over time while no significant effect on diameter and tube wall thickness until a steady-state situation occurs [67, 85, 86]. However, due to the decrease of the F − concentration in the electrolyte, where the ion transport rate decreased, the growth rate of nanotubes is reduced. After reaching a stable condition between tube growth at the bottom and chemical/electrochemical dissolution at the top, we will find no further increase in length of the nanotubes [87]. As time continues to go, pipe orifice becomes an irregular polygon resulting in TiO2 spikes and coverings which can be seen on the surface of the TiO2 nanotube arrays [36]. It is worth mentioning that enlightened by the success of aluminum-repeated anodization for self-organized porous alumina [88], the two-step anodization of titanium for such a highly ordered hexagonally packed nanostructure of titania has appeared [43, 77, 89,90,91]. After the first-step anodization, the first nanotube layer from the Ti foil should be removed ultrasonically or by using an adhesion tape which leads to a surface where the remaining Ti is covered by comparably ordered dimples. Researches have shown that the former treatment helps to avoid potential mechanical damage to the Ti surface and also improve the structural uniformity of the TiO2 nanotubes to a great extent [77, 90, 91]. In the second anodization step, the pretreated Ti foil would be used as anode again with or without changes in parameters of oxidation conditions. It is subsequently found that the highly ordered and vertically oriented titania nanotubes, have greater potential in such fields as photocatalysis [77], photoelectriochemical activity [92, 93], and biological interaction with cells [94] than the disordered nanotubular titania.
Electrolyte Temperature
Temperature restricts the growth and quality of titania nanotube arrays, directly affecting the rate of oxide growth, length, and wall thickness of the structure [64, 95]. Wang and Lin first reported the effect of electrolyte temperature in both aqueous and non-aqueous electrolyte on anodic oxidation of titanium [82]. In aqueous electrolyte, with the temperature increasing, a slight diminish in the internal diameters was observed while the external diameters remained the same [68]. The reason may be the dissolution induced by electrical field and fluoride ions are similar while the oxide formation rate is higher than that at lower temperature. In non-aqueous electrolyte containing fluoride ions, the outer nanotube diameter was found to be largely increased by the increasing electrolyte temperature [82]. This may be because at lower temperature, the ion mobility of fluorine in some viscous electrolyte is further inhibited, leading to much slower dissolution of newly formed titania, which subsequently lead to a smaller nanotube diameter. As chemical dissolution rate increases, surface of TiO2 nanotubes arrays can easily produce excessive corrosion, resulting in lodging nanotubes and agglomeration. Therefore, the appropriate bath temperature for stable TiO2 nanotube arrays is at room temperature [82, 95, 96].
Modification of Nanotubes Properties
Increasing applications of TiO2 nanotubes as a novel semiconductor are closely related to its photoelectriochemical (PEC) performance; however, they are sometimes prevented by two fundamental drawbacks:(I) the wide band gap (3.0 eV for the rutile phase and 3.2 eV for the anatase phase) can only absorb ultraviolet light, which accounts for less than 10% of the sunlight [97], resulting in low average utilization ratio of solar energy and (II) the low electrical conductivity cannot efficiently transfer photogenerated carries. At the same time, the photoelectrons and vacancies can be easily recombined, thus making low electron mobility rate or quantum confinement effects [98]. Hence, post-treatment of TiO2 nanotubes is the key to improve the performance of its materials and related devices successfully. Considerable researches have been reported on modified methods to reduce the recombination of photogenerated electron-hole pair rate, speed up the electron transfer rate, and enhance the photoelectriochemical activity of TiO2 nanotubes. The research of the methods for the improvement of the photoelectriochemical properties of TiO2 nanotubes will be reviewed, including thermal annealing, doping, and surface modification. As for promising modification in biomedical fields, we will present in the application section.
Thermal Annealing
The crystallinity of the nanotube arrays and their conductivity, lifetime of charge carrier, and photoresponse depend mainly on the thermal annealing temperature and atmosphere [99, 100]. The as-prepared TiO2 nanotubes above are amorphous in nature but can be annealed to anatase or rutile phase, or mixtures of both phases relying on the specific temperature [1, 3, 40, 92, 100]. It is demonstrated that amorphous nanotube layers grown in a glycerol-based electrolyte containing fluoride ions have low photocurrents and an incident photon-to-electron conversion efficiency (IPCE) below 5% due to lots of structural defects while anatase phase nanotubes exhibit an IPCE value up to 60% thus attracting more interest to applications such as dye-sensitized or perovskite solar cells [93]. As well in mixed water-glycerol electrolyte with F − , Das et al. stated their points that if the self-organized TiO2 nanotube arrays with thickness about 1 μm were annealed around 300–500 °C, the anatase phase of TiO2 as the most preferred crystalline structure could be observed. The single anatase structure of nanotubes with the best photoelectriochemical properties and the lowest resistivity could be fabricated when annealed at 400 °C. At temperature higher than 600 °C, a track of typical rutile appeared and with a further increase in annealing temperature the percentage and quality of the rutile phase increased [92]. It should be noted that in Jaroenworaluc’s work, rutile phase was detected in anodic nanotube layers grown in aqueous NaF/Na2 SO4 with thickness of approximately 1.5 μm at 500 °C heat treatment and became the dominant phase at 600 °C. Whereas at 550 °C, partial nanotubes began to break down [101]. It begins to cause the collapse of the entire nanostructure formed in aqueous NaF/Na2 SO4 with the continuous increase of temperature (800–900 °C) or the extended annealing time [3]. While for extended temperature, the crystalline structure of the nanotubes completely converts to rutile phase at above 900 °C [3]. Some researchers demonstrated a loss of the typical single-walled nanotube layers morphology when the annealed temperature rose above 580 °C [102]. Besides the whole annealing process especially the heating rate controls, the morphological structures of the entire nanotube arrays [40]. The double-walled nanotube layers prepared from ethylene glycol (containing less than 0.2 wt% H2 O), with the addition of HF and H2 O2 , have such a high stability that can keep their structure intact until temperature is higher than 900 °C with a heating rate of 1 °C s −1 . However, the double-walled nanotubes begin to collapse as soon as the temperature reaches 500 °C when the heating rate is 25 °C s −1 . Most extraordinarily, with the high speed of 50 °C s −1 the entire separated nanotubes fuse into a highly ordered porous membrane [40]. Xiao et al. obtained crystallized titania nanotubes arrays with calcination in different gases like dry nitrogen, air, and argon indicating nanotubes in dry nitrogen appeared to have enhanced electrochemical and photoelectrical properties who also found out that with the increasing temperature internal diameter decreased while wall thickness increased at the expense of nanotubes length [103].
As shown in Fig. 8, the conductivity along the TiO2 nanotubes with three different thickness is strongly affected by annealing temperature. Smallest resistance is observed at about 350–450 °C when the amorphous nanotube arrays are totally converted into anatase layers [99]. And it is evident to see that specific resistivity increases with thicker nanotube arrays which can be shown more clearly in the inset in Fig. 8. Furthermore, calcination temperature is responsible for the decrease in the length of the anatase TiO2 nanotubes. As shown in Fig. 9a, increment of temperature between 300 and 500 °C causes the as-prepared nanotube arrays slightly changing in thickness from 13.6 to 12.6 μm. When annealing temperature continuously increases to 600 °C, the average length of the nanotubes decrease dramatically to 6.6 μm. Figure 9b shows conversion from anatase TiO2 to rutile phase TiO2 occuring at 500 °C when the rutile barrier layer is formed on the bottom of the TiO2 nanotube arrays along the anatase nanotubes by consuming the bottom layer if the annealed temperature is further increased. This leads to a length decrease and corresponding photocatalytic activity decline [104].
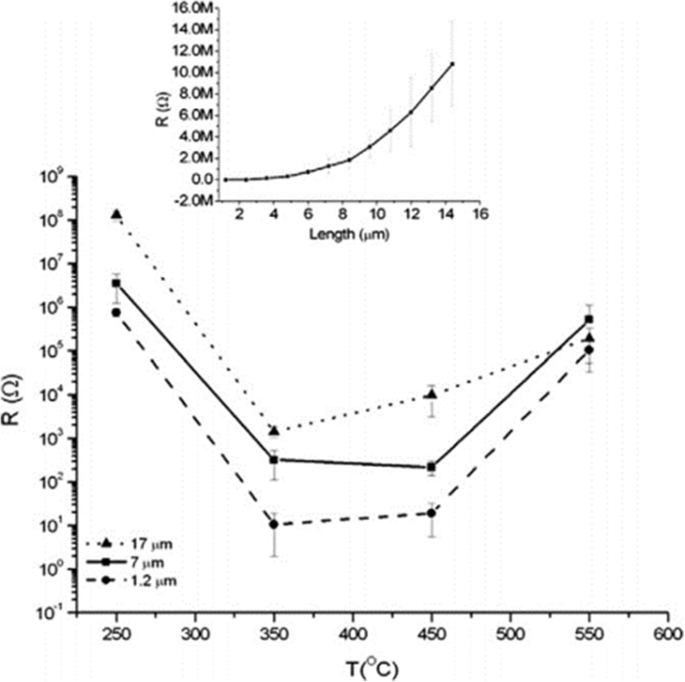
Electrical resistance as a function of the annealing temperature for the different nanotube layer thicknesses. The curve shows electrical resistance measurement for different titania nanotube arrays grown in ethylene glycol based electrolyte containing HF and water at different temperature and the influence of thickness on resistance. The inset shows more details about the relationship between the thickness of the nanotube arrays annealed at 250 °C and their specific resistivity. Reproduced from ref. [99]
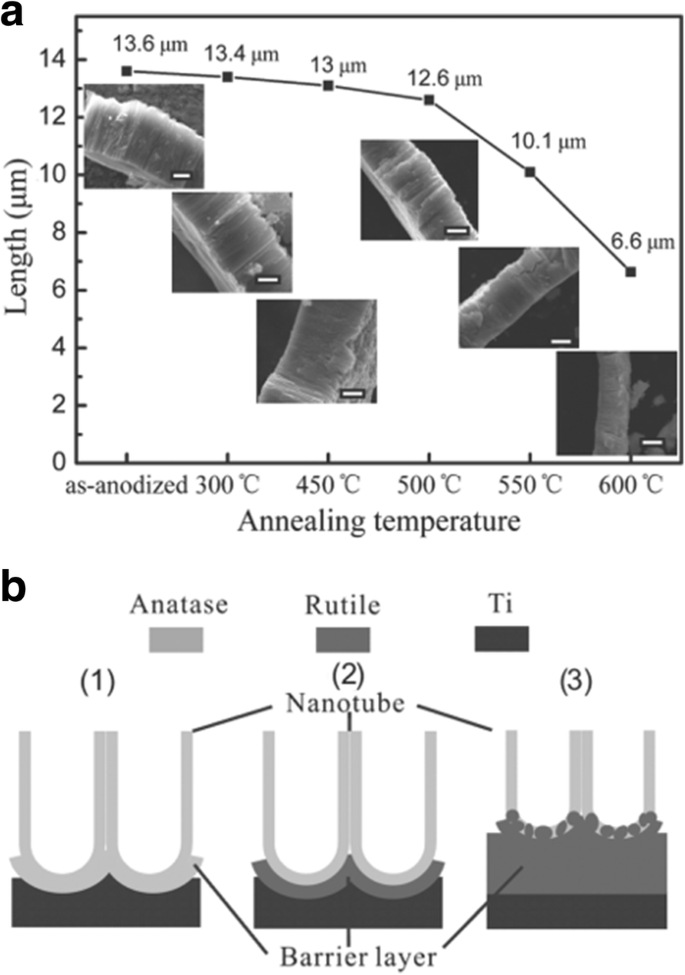
Evolution of titania nanotube arrays at different calcination temperatures. The electrolyte was ethylene glycol containing 0.3 wt% ammonium fluoride and 5 vol% distilled water. een The decrease in the thickness of titania nanotube arrays at different annealing temperature from 300 to 600 °C. The insets are corresponding SEM images and the scale bar is 5 μm. b The schematic of crystallization process of anodic titania nanotubes annealed at (1) 450 °C, (2) 500 °C, and (3) 600 °C in air. Reproduced from ref. [104]
Doping
Doping ions or atoms into titania lattice, a substitution within the lattice either at Ti 4+ or O 2− sites, on the one hand, changes the lattice constants and bond energy. On the other hand, it is beneficial to the separation between photogenerated electron and hole pair, which in turn adjusts the band gap and improves the photoelectrochemical performance of nanotubes [15]. The impurity doping has been commonly applied to extend the light absorption onset of TiO2 nanotubes by either introducing subbandgap states or adjusting its bandgap width [105]. Lately, co-doping approach has been proposed as a more efficient way to reduce the band gap and adjust energy band level in favor of photoelectriochemical reactions [106, 107]. There are various kinds of doped-elements and preparation methods, and Table 2 summarizes some methods and the doping effects of doped titania nanotubes.
The most typical doped TiO2 nanotubes are as follows:
- i.
Metal-doped TiO2 nanotubes such as Nb [107], Fe [108], Cu [109], Cr [110], Zr [111], Zn [112], and V [113]
- ii.
Non-metal-doped TiO2 nanotubes such as N [105], F [114], B [115], C [116], S [117], and I [118]
- iii.
Co-doped TiO2 nanotubes such as N–Ta [105], N–Nb [107], and C–N–Ni [119]
Choiet systematically studied the photoreactivities of 21 metal ion-doped quantum-sized TiO2 doping with Fe, Mo, Ru, Os, Re, V, and Rh significantly increases quantum efficiency, while Co and Al doping decreases the photoreactivity [120]. Momeni et al. recently obtained Fe-TiO2 nanotube (Fe-TNT) composites using different amounts of irons to decorate anodically formed TiO2 nanotubes with potassium ferricyanide as the iron source, indicating that Fe doping efficiently accelerates the photocatalytic performance for water splitting [108]. Not limited to transition metals, other elements including N [105], F [114], B [115], C [116], S [117], and I [118] are successfully explored. Nitrogen-doped TiO2 nanotubes turns out to be a promising path to narrow the band gap energy with enhanced photocurrent response in the visible light and the tube length influences the magnitude of conversion efficiency [121, 122]. Kim and co-workers proved that TaOxNy layer-decorated N-TNT (N-doped TiO2 nanotubes) as dual modified TNTs have significantly improved both visible (3.6 times) and UV (1.8 times) activities for water splitting [105]. At present, more researches are aimed at co-doping which exhibits remarkable synergistic effect causing a significant improvement on photoelectriochemical properties. Chai et al. grew Gd–La co-doped TiO2 nanotubes by an ultrasonic hydrothermal method, enhancing visible light photocatalysts [123]. Cottineau et al. modified titania nanotubes with nitrogen and niobium to achieve co-doped nanotubes with noticeably enhanced photoelectriochemical conversion efficiency in the visible light range [107]. Nevertheless, the mechanism for increasing photoconductivity and synergistic effect of various elements on co-doping remains a further study.
Surface Modification
Surface modification means decoration on surface of TiO2 nanotube arrays with nanoparticles (metal, semiconductors, and organic dyes). Nanowire arrays can also be fabricated by electrodeposition into titanium oxide nanotubes [124]. TiO2 nanotube is a semiconductor with a wide band gap, which can only absorb ultraviolet light [97, 125]. Any other nanomaterials which possess a narrow band gap or can absorb the visible light can be used as a sensitizer for titania nanotubes. Silver nanoparticles can be decorated on the tube wall by soaking the titania nanotube arrays in AgNO3 solutions and photocatalytically reducing Ag + on a TiO2 surface by UV illumination [126]. Ag/TiO2 nanotubes show a significantly higher photocatalytic activity and good biological performance compared with neat TiO2 nanotubes [126, 127]. Some compositions such as graphene oxide GO [128], CdS [129], CdSe [130], and ZnFe2 O4 [131]. can be modified on TiO2 nanotube arrays. Lately, GO have attracted much scientific interest in nanoscale devices and sensors which is easy to combine with nanostructure materials to compose some compounds. Titania nanotubes fabricated by anodization in water-ethylene glycol electrolyte consisting of 0.5 wt% ammonium fluoride (NH4 F) can be incorporated with GO by cyclic voltammetric method, which achieve higher photocatalytic activity and more effective conversion efficiency (GO-modified vs pure nanotubes =26.55%:7.3%) of solar cell than unmodified TiO2 nanotubes [128]. Semiconductor composite is a method improving the performance of titania nanotubes via, in some specific way, combining two kinds of semiconductors with different band gap [132]. Yang et al. decorated CdSe nanoparticles on the surface of TiO2 nanotubes by applying an external electric field to accelerate CdSe nanoparticles in nanochannels resulting in a material with more stable and higher photoresponse to visible light. Furthermore, the degeneration rate of anthracene-9-carbonxylic acid when exposed to the green light irradiation indicating that CdSe dominates the photocatalytic process under visible light [130].
Besides, other oxide nanoparticle deposition such as WO3 [133] or TiO2 [134] onto TiO2 nanotubes by the hydrolysis of a chloride precursor also turns out to augment the surface area and improve the solar cell efficiency. Another very effective approach is to consider organic dyes as sensitizers for TiO2 nanotubes to improve its optical properties [135]. Lately, atomic layer deposition (ALD) becomes an established procedure to modify TiO2 nanotube layers. ALD appears to be a very uniform and precisely controllable deposition process to functionalize nanotubes in conformably coating the surface of the nanotube layers with one atomic layer after another of a secondary material, such as Pd [136], ZnO [137], Al2 O3 [138], CdS [139], or TiO2 [140].
Biomedical Applications
Historically, the mentioned milestones were reported on the fabrication of titania nanotube arrays contributing to widen the promising applications over the past 20 years in the areas ranging from anticorrosion, self-cleaning coatings, and paints to sensors [141,142,143], dye-sensitized and solid-state bulk heterojunction solar cells [144,145,146], photocatalysis [147, 148], eletrocatalysis, and water photoelectrolysis [149, 150]. They also outperform in biomedical directions as biocompatible materials, toward biomedical coatings with enhanced osseointegration, drug delivery systems, and advanced tissue engineering [15, 135, 141, 142, 151]. In the following section, we will give an overview of current efforts toward TiO2 nanotubes biomedical applications. Titania nanotubes possess good biocompatibility as they show some antibacterial property, low cytotoxicity, good stability, and cytocompatibility including promoting adhesion, proliferation, and differentiation of osteoblast and mesenchymal stem cells (MSCs) with a high surface area-to-volume ratio and controllable dimensions [152,153,154,155].
However, Ti products have inadequate antibacterial ability and efforts have been made to improve their antibacterial properties such as modifications on titania nanotubes for biomedical applications like bioimplant [126, 156].
Biological Coatings And Interactions with Cells
A number of in vitro and in vivo studies have demonstrated that MSCs, osteoblasts and osteoclasts show size-selective response which means the effect of size holds an important position in cell interaction where the optimized size for cell adhesion, proliferation, growth, and differentiation is ranging from 15 to 100 nm [153, 157, 158]. Particularly, it was demonstrated that the TiO2 nanotubes with a diameter of 70 nm was the optimal nanoscale geometry for the osteogenic differentiation of human adipose-derived stem cells (hASCs) [159]. Smith et al. reported increased dermal fibroblasts and decreased epidermal keratinocyte adhesion, proliferation, and differentiation on TiO2 nanotube arrays (diameter 70–90 nm, length 1–1.5 μm) [160]. As shown in Fig. 10, Peng et al. found that nanotubular surface preferentially promoted proliferation and function in endothelial cells (EC) while decreased in vascular smooth muscle cell (VSMC) by measuring EdU, a thymidine analog which is incorporated by proliferating cells [161]. Furthermore, it is pointed out that surface wettability of the TiO2 nanotube layers is recognized as a critical factor for cell behavior which can be adjusted by changing the diameter of the nanotubes. That is to say, water contact angles can be altered without changing the surface chemistry [158]. To get further understanding of the effect of TiO2 nanotube layers to bone-forming cells as well as stem cells response, Park et al. seeded green fluorescent protein-labeled rat MSCs on TiO2 nanotube layers with six different diameters (15, 20, 30, 50, 70, and 100 nm), resulting in cell activity that is sensitive to nanoscale surface topography with a maximum in cell activity obtained for tube diameters of approximately 15–30 nm. Such lateral spacing exactly corresponds to the predicted lateral spacing of integrin receptors in focal contacts on the extracellular matrix, forcing clustering of integrins into the closest packing, resulting in optimal integrin activation. While tube diameters larger than 50 nm, severely impaired cell spreading, adhesion, and spacing of 100 nm may lead to the cell apoptosis [94]. Besides adjusting the size of the nanotubes, surface modification loaded with bioactive factors should be highlighted, in which case biomedical properties can be further optimized. In the case of bone implants, hydroxyapatite (HA) formation is important for osseointegration. Recent works have shown hydroxyapatite nanocrystalline coating onto the nanotubular TiO2 results in further enhanced osseointegration with strong adhesion and bond strength, and a drastic enhancement of deposition rate is observed [162, 163]. Nanotubular TiO2 surface can greatly enhance the natural apatite growth rate in simulated body fluid (SBF) compared with flat surfaces [10, 164]. The alkaline-treated TiO2 nanotubes with NaOH solutions are more bioactive in SBF, where sodium titanate can significantly accelerate nucleation and the growth of HA formation presenting a well-adhered bioactive surface layer on Ti due to its larger surface area and promoted mechanical interlocking between HA and TiO2 nanotubes [165, 166]. Electrodeposited with hydroxyapatite, higher adhesion of TiO2 nanotubes has been described in the literature by means of adhesive tape test and the live/dead cell staining study which is essential for early bone formation [166]. The results also showed that at the length of 560 nm the highest adhesion of HA surface on the nanotubes is observed. Also the nanotube surface can indeed strengthen Collagen type I expression in vivo experiment which is considered to be a basic initial bone matrix protein in bone formation [167]. Moreover, annealing of the amorphous nanotubes to anatase or a mixture of anatase and rutile was found to be an important factor in the apatite formation process [164].
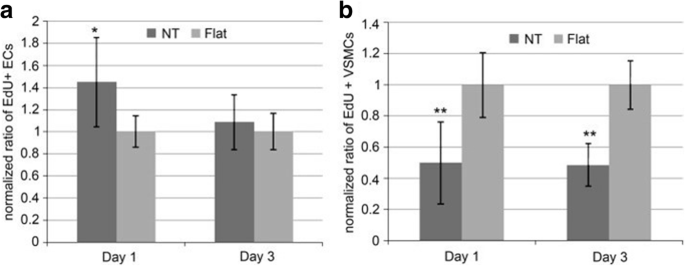
Ratio of EdU positive a ECs and b VSMCs on flat or nanotube substrate. It is normalized by the average proportion of positive cells on flat surfaces on day 1 and 3. Data is presented as average ± standard deviation. *p < 0.05, **p < 0.01 versus same day flat control, n = 6 reproduced from ref. [161]
Drug Delivery and Antibacterial Ability
Furthermore, the tubular nature of TiO2 in biomedical devices may be exploited as gene and drug delivery carriers with living matter due to its high surface area, controllable pore, and self-ordered structure [1, 15]. When the orthopedic bioimplant is placed into the bone defect, persistent and chronic infection is one of the most common and serious complications associated with biomedical implantation [16, 168]. Certain dimension and crystallinity may be useful to prevent bacteria adhesion and promote bone formation. The thermal annealing has decreased the number of bacteria adhering to the Ti surface. It could be in part because heat treatment removes the fluorine content which has a tendency to attract bacteria. The research also indicates that nanotubes with 60 or 80 nm in diameter decrease the number of live bacteria as compared to lower diameter (20 or 40 nm) nanotubes [169, 170].
Bauer et al. loaded epidermal growth factor (EGF) and bone morphogenetic protein-2(BMP-2) onto the TiO2 nanotubes surface by covalent attachment. They observed positive influence on the behavior of MSCs on 100-nm nanotube arrays where cell count was at much higher levels compared to the untreated one [171]. Lately, titania nanotubes loaded with antibiotics contribute to suppressing bacterial infections. As gentamicin sulphate (GS) is mostly widely used with highly water solubility, Feng et al. loaded titania nanotubes with GS through physical adsorption and cyclic loading which can treat many types of bacterial infections [172]. Zhang et al. fabricated titania nanotubes loaded with vancomycin to investigate the increasing biocompatibility and obvious antibacterial effect on Staphylococcus aureus [173]. However, systemic antibiotics in clinical will bring many side effects. The release of antibiotics from the nanotubes is too fast to maintain the long-term antibacterial ability, and the use of antibiotics may develop resistant strains [126, 168, 174]. Ensuring a constant release rate becomes a crucial but difficult part in the field of drug delivery. In strategies like surface modification, controlling the dimension of nanotube arrays, biodegradable polymer coating have been employed to solve the issue [21]. Drug release of several drugs such as antibiotics or growth factors from titania nanotube arrays can be adjusted by varying their diameters and lengths [152, 175, 176]. Feng et al. covered a thin film comprising a mixture of GS and chitosan on GS-loaded titania nanotubes and showed a controlled release of the drug providing sustained release effects to a certain extent [172]. Titania nanotube arrays as drug nanoreservoirs on Ti surface for loading of BMP-2 were fabricated by Hu et al. and then further covered with gelatin/chitosan multilayers to control the release of the functional molecule meanwhile maintain the bioactivity for over 120 h via a spin-assisted layer-by-layer assembly technique which is mainly based on electrostatic interactions between polyanions and polycations as well as promote osteoblastic differentiation of MSCs [177]. Lai et al. successfully fabricated Chi/Gel multilayer on melatonin-loaded TiO2 nanotube arrays to control the sustained release of melatonin and promote the osteogenic differentiation of mesenchymal stem cells [178]. Karan et al. synthesized titania nanotubes loaded with the water-insoluble anti-inflammatory drug indomethacin and modified lactic-co-glycolic acid on surface as a polymer film in order to extend the drug release time of titania nanotubes and produce favorable bone cell adhesion properties, with reduced burst release (from 77 to> 20%) and extended overall release from 4 days to more than 30 days [152]. As previous study reported that surface treatment of implants with N -acetyl cysteine (NAC) may reduce implant-induced inflammation and promote faster bone regeneration [179], Lee et al. examined the feasibility of N -acetyl cysteine-loaded titania nanotubes as a potential drug delivery system onto an implant surface, and the data indicates the enhanced osseointegration and the value of the small animal model in assessing diverse biological responses to dental implants. Besides, TiO2 nanotube arrays are suitable for loading inorganic agents like Ag, Sr, and Zn to obtain long-term antibacterial ability and osseointegration [126, 180,181,182]. Ag nanoparticles have been incorporated into TiO2 nanotube arrays previously with satisfactory small possibility to develop resistant strains, a broad-spectrum antibacterial property, low cytotoxicity, and good stability by immersion in a silver nitrate solution followed by ultraviolet light radiation [126]. Zhang et al. demonstrated that a series of porous TiO2 coatings with different concentrations of silver had significant inhibition effect on Escherichia coli en Staphylococcus aureus . Besides, only with the optimum amount of silver can the coatings retain the antibacterial effect but without any measurable cytotoxicity to cells [183]. Due to cytotoxicity observed by the excessive release of Ag + subsequently, titania nanotube arrays with Ag2 O nanoparticles embedded in the wall are prepared on Ti by TiAg magnetron sputtering and anodization in order to get slower and more controllable silver ion release [184]. That is because the TiO2 barrier is surrounded thereby minimizing the cytotoxicity induced by burst or large Ag + release.
Similar to Ag, Zn possesses antibacterial and anti-inflammation properties, and osteogenesis induction [185,186,187]. Huo et al. produced anodic TiO2 nanotube arrays at 10 V and 40 V (NT10 and NT40) incorporated with Zn by hydrothermal treatment at 200 °C for 1 and 3 h (NT10-Zn1, NT10-Zn3, NT40-Zn1, and NT40-Zn3) in Zn containing solutions, followed by annealing at 450 °C for 3 h in air. NT40-Zn3 has the largest Zn loading capacity and releases more Zn compared with other samples. The amounts of Zn released diminish gradually with time and nearly no Zn can be detected 1 month later except sample NT40-Zn3 (Fig. 11). The NT-Zn samples present different antibacterial ability. It is evident that NT40-Zn3 and NT10-Zn3 effectively kill more adherent bacteria as well as surrounding planktonic bacteria in the early stage. Figure 12a describes a synergistic effect of both released and surface incorporated Zn while Fig. 12b explains the effect of the released Zn [181].
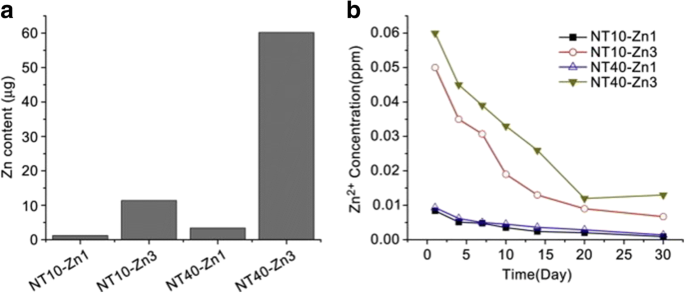
een Total amounts of Zn incorporated into the NT-Zn samples for the 1 cm 2 coatings and b non-cumulative Zn release profiles from NT-Zn into PBS. Reproduced from ref. [181]
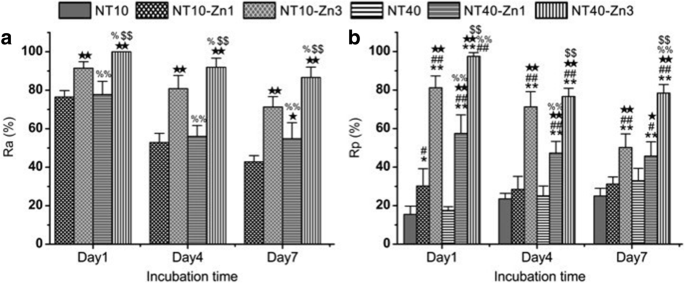
een Antibacterial rates versus adherent bacteria on the specimen (Ra) and b antibacterial rates against planktonic bacteria in the medium (Rp) *, **p < 0.05 and 0.01 vs NT10; # , ## p < 0.05 and 0.01 vs NT40; ★ , ★★ p < 0.05 and 0.01 vs NT10-Zn1; % , %% p < 0.05 and 0.01 vs NT10-Zn3; $ , $$ p < 0.05 and 0.01 vs NT40-Zn1. Reproduced from ref. [181]
Conclusions
This review presents the historical developments and traditional formation mechanism of titania nanotube arrays grown by electrochemical anodization as well as the approaches to influence and modify morphology in order to improve their performances. We also focus on current efforts toward TiO2 nanotubes applications in biomedical directions. Those steady progresses have demonstrated that TiO2 nanotubes are playing and will continue to play an important role in material science, but there are still some aspects needed to be further improved.
- 1.
The synthesis of TiO2 nanotube arrays is already comparatively mature so far in fact, but how to simplify the technology for the purpose of large-scale production in industry with extending practical operability and how to precisely control nanotube geometry efficiently by varying the anodic parameters so as to obtain optimized properties have yet to be further investigated.
- 2.
The formation mechanisms of anodic TiO2 nanotubes have gradually become a hotspot of research due to their unique structure and excellent performances but the exact mechanism remains controversial. Conventional FAD explains the growth process and the porous structure of TiO2 nanotubes, but the combination of viscous flow model and growth model of two currents can give a comprehensive explanation to the growth process. Notably, the validity of oxygen evolution resulting from electronic current has much room for investigation.
- 3.
Modification is key for improving performances of titania nanotube arrays. Thus, we need to explore more methods for modification and take full advantage of the self-organized nanostructure. Through self-assembling inorganic, organic, metallic, and magnetic nanoparticles into or onto the tubes as nanocomposites with broad spectral response to visible light, high quantum efficiency, and stabilizing properties, applications could be widened. Currently, ALD appears to be an option to coat the titania nanotube layers homogenously and precisely from the bottom to the tube mouth, resulting in many advanced functionalities of the newly prepared nanotube layers. Nevertheless, further optimization of the ALD process toward coatings and inner fillings is demanded.
- 4.
TiO2 nanotube researches in biomedical directions are still in their infancy and have a long distance to go in clinical use. The biological reaction between cells and titania nanotubes has to develop from cellular level to molecular level and from morphological changes to molecular alterations. It has been shown that adhesion, spreading, and growth of osteoblast and mesenchymal stem cells strongly depends on nanotube diameter, so the regularity and principle of this phenomenon as well as other factors affecting cells’ behaviors need to be further explored.
Afkortingen
- ALD:
-
Atoomlaagafzetting
- BMP-2:
-
Bone morphogenetic protein-2
- DMSO:
-
Dimethyl sulfoxide
- DSSCs:
-
Kleurstofgevoelige zonnecellen
- EBSD:
-
Electron Backscatter Diffraction
- EG:
-
Endothelial cells
- EdU:
-
A thymidine analog
- EGF:
-
Epidermale groeifactor
- FAD:
-
Conventional field-assisted dissolution
- FE-SEM:
-
Veldemissie scanning elektronenmicroscopie
- Fe-TNTs:
-
Fe-doped TiO2 nanotubes
- FIB:
-
Focused ion beam
- GO:
-
Grafeenoxide
- GS:
-
Gentamicin sulphate
- HA:
-
Hydroxyapatite
- hASCs:
-
Adipose-derived stem cells
- IPCE:
-
Incident photon-to-electron conversion efficiency
- MSC's:
-
Mesenchymale stamcellen
- NAC:
-
N -Acetyl cysteine
- N-TNT:
-
N-doped TiO2 nanotubes
- PEC:
-
Photoelectriochemical
- SBF:
-
Simulated body fluid
- UV:
-
Ultraviolet
- VSMC:
-
Vascular smooth muscle cell
Nanomaterialen
- 4 Gebruik van Hafnium | De toepassingen van Hafnium- en Hafniumlegeringen
- Multifunctionele gouden nanodeeltjes voor verbeterde diagnostische en therapeutische toepassingen:een overzicht
- Vooruitgang en uitdagingen van fluorescerende nanomaterialen voor synthese en biomedische toepassingen
- Grafeen- en polymeercomposieten voor toepassingen met supercondensatoren:een recensie
- Op weg naar TiO2-nanovloeistoffen - Deel 2:Toepassingen en uitdagingen
- Het effect van contactloos plasma op structurele en magnetische eigenschappen van Mn Х Fe3 − X О4 Spinels
- TiO2-nanobuisarrays:gefabriceerd op basis van een zacht-hard sjabloon en de korrelgrootte-afhankelijkheid van veldemissieprestaties
- Vergelijkende studie van de elektrochemische, biomedische en thermische eigenschappen van natuurlijke en synthetische nanomaterialen
- Een gemakkelijke methode voor het laden van CeO2-nanodeeltjes op anodische TiO2-nanobuisarrays
- Freesspil en de opmerkelijke toepassingen
- 9 soorten draadsnijgereedschappen en de toepassingen