Afdrukbare, zeer gevoelige flexibele temperatuursensoren voor bewaking van de lichaamstemperatuur:een overzicht
Abstract
In de afgelopen jaren zijn de ontwikkeling en het onderzoek van flexibele sensoren geleidelijk verdiept en zijn de prestaties van draagbare, flexibele apparaten voor het bewaken van de lichaamstemperatuur ook verbeterd. Voor het menselijk lichaam weerspiegelen veranderingen in lichaamstemperatuur veel informatie over de menselijke gezondheid, en abnormale veranderingen in lichaamstemperatuur duiden meestal op een slechte gezondheid. Hoewel de lichaamstemperatuur onafhankelijk is van de omgeving, wordt de temperatuur van het lichaamsoppervlak gemakkelijk beïnvloed door de omgeving, wat een uitdaging vormt voor apparatuur voor het bewaken van de lichaamstemperatuur. Om realtime en gevoelige detectie van verschillende delen van de temperatuur van het menselijk lichaam te bereiken, hebben onderzoekers veel verschillende soorten zeer gevoelige flexibele temperatuursensoren ontwikkeld, de functie van elektronische huid geperfectioneerd en ook veel praktische toepassingen voorgesteld. Dit artikel bespreekt de huidige onderzoeksstatus van zeer gevoelige flexibele temperatuursensoren met patronen die worden gebruikt om veranderingen in lichaamstemperatuur te bewaken. Eerst zijn veelgebruikte substraten en actieve materialen voor flexibele temperatuursensoren samengevat. Ten tweede worden patroonfabricagemethoden en -processen van flexibele temperatuursensoren geïntroduceerd. Vervolgens worden flexibele temperatuurwaarnemingsprestaties uitgebreid besproken, inclusief temperatuurmeetbereik, gevoeligheid, responstijd, temperatuurresolutie. Ten slotte wordt de toepassing van flexibele temperatuursensoren op basis van zeer delicate patronen gedemonstreerd en zijn de toekomstige uitdagingen van flexibele temperatuursensoren naar voren gekomen.
Inleiding
Alle levensactiviteiten van het menselijk lichaam op basis van stofwisseling en relatief constante lichaamstemperatuur zijn noodzakelijk voor een gezonde stofwisseling [1]. Hyperthermie of hypothermie zal de activiteit van enzymen in het lichaam beïnvloeden, waardoor de normale werking van het menselijke metabolisme wordt aangetast, waardoor aandoeningen van verschillende cellen, weefsels en organen ontstaan, en in ernstige gevallen zelfs de dood. Het kan zien dat de relatieve stabiliteit van de lichaamstemperatuur een noodzakelijke voorwaarde is voor het handhaven van een stabiele omgeving in het lichaam en het verzekeren van de regelmatige voortgang van levensactiviteiten zoals metabolisme. Voor het menselijk lichaam weerspiegelen veranderingen in lichaamstemperatuur veel informatie over de menselijke gezondheid, en abnormale veranderingen in lichaamstemperatuur duiden meestal op een slechte gezondheid. Bij het monitoren van de menselijke gezondheid [2, 3] is lichaamstemperatuur een essentiële factor die niet kan worden genegeerd, en realtime en nauwkeurige monitoring van de lichaamstemperatuur is bijzonder belangrijk.
Flexibele temperatuursensoren hebben zich ontwikkeld in de richting van draagbare, zeer gevoelige, draagbare, grote, nauwkeurige en realtime trends. De flexibele temperatuursensor gebruikt voornamelijk de elektrische signaalverandering van het thermogevoelige materiaal als gevolg van de temperatuurverandering om de realtime bewaking van de temperatuur te realiseren [4]. Het gebruikt ook het karakter van het flexibele substraat om zich strikt aan de huid te hechten om zijn functie te realiseren. Vergeleken met traditionele temperatuurmeetinstrumenten, zijn er niet alleen moeilijk te dragen, duur en toepasbaar bij bewakingsgelegenheden, maar zijn er ook beperkingen vanwege de opzettelijke of onopzettelijke beweging van de patiënt en het onvermogen om specifieke locaties (zoals wonden) te bewaken [5 ], tumorablatieplaatsen in het lichaam [6]), kunnen gemakkelijk leiden tot onnauwkeurige of onvolmaakte meetresultaten. Om de bovenstaande problemen op te lossen, zijn draagbare, flexibele, dunne en gevoelige temperatuursensoren met patronen een onderzoekshotspot voor wetenschappelijke onderzoekers geworden.
De afgelopen jaren is het onderzoek naar flexibele temperatuursensoren voor het bewaken van de lichaamstemperatuur voortdurend in ontwikkeling geweest en zijn er veel innovaties [7]. Het gebruik van patroonfabricage om flexibele temperatuursensoren op grote oppervlakten te fabriceren is een ontwikkelingstrend geworden [8]; het nabootsen van biologische structuren in de natuur is een uitstekend idee [9]. De octopuspoten met adsorptie-eigenschappen, de wangen van de adder die veranderingen in biologische temperatuur kunnen waarnemen [10], en de snorhaarachtige structuren [11] van sommige geleedpotigen of zoogdieren hebben ook een temperatuurgevoelige functie; om de lichaamstemperatuur die door de sensor wordt bewaakt duidelijk weer te geven, zullen de onderzoekers de flexibele temperatuursensor in een array plaatsen [12,13,14], en het beeldvormingsapparaat of het elektrochrome materiaal ervan kan gebruiken om de warmtebeeldkaarten te visualiseren [5, 15] , 16]. Diepgaand onderzoek naar flexibele temperatuursensoren biedt ook passende technische ondersteuning om aan hoge eisen te voldoen, zoals een zeer gevoelige bewaking van de lichaamstemperatuur.
Dit artikel bespreekt de recente onderzoeksvooruitgang van hooggevoelige flexibele temperatuursensoren bij het bewaken van de lichaamstemperatuur, warmtegevoelige materialen, productiestrategieën, basisprestaties en toepassingen. Het eerste deel zal materialen selecteren voor flexibele temperatuursensoren en een verscheidenheid aan flexibele substraten samenvatten, warmtegevoelige materialen die kunnen worden gebruikt als flexibele temperatuurbewakingssensoren. Het tweede deel richt zich op het gebruik van flexibele temperatuursensoren in de literatuur van de afgelopen jaren. De fabricagemethode met patronen wordt herzien, waarbij het typische productieproces wordt weergegeven. Het derde deel introduceert de kritische prestatieparameters van temperatuursensoren. Het vierde deel toont de toepassingsscenario's en praktische toepassingen van flexibele temperatuursensoren in de afgelopen jaren. Ten slotte worden de potentiële uitdagingen en toekomstige ontwikkelingsperspectieven van bedrukbare, zeer gevoelige flexibele temperatuursensoren kort besproken.
Methoden
Materiaal
Flexibele substraten
In de afgelopen jaren is de toepassing en het onderzoek van flexibele materialen op het gebied van elektronische technologie en medisch en gezondheid geleidelijk toegenomen. De fabricage van flexibele sensoren vereist dat de sensor zelf flexibel, rekbaar en ductiel is en de substraten en circuits waarvan hij afhankelijk is. Specifieke rek- en rekeigenschappen om zich aan te passen aan de hechting op het menselijk lichaamsoppervlak, gewone flexibele substraten worden meestal verwerkt tot een film, zoals polydimethylsiloxaan (PDMS) [17,18,19,20], polyimide (PI) [21, 22 ], polyurethaan (PU) [23], polyethyleentereftalaat (PET) [24, 25], polyvinylalcohol (PVA) [26], polyvinylbutyral (PVB) [27], papier [28, 29], siliconenrubber [5 , 30, 31] en huidvriendelijkere biologisch afbreekbare materialen kunnen ook worden gebruikt, zoals pectine [32], katoen, zijde [33] en andere cellulosematerialen [34, 35].
Op dit moment is het meest gebruikte flexibele substraat in flexibele temperatuursensoren PDMS, een uitstekend thermisch en elektrisch isolatiemateriaal, met een relatieve permittiviteit van 2,3-2,8 en een volumeweerstand van \({1}{\text{.2}) } \times {10}^{{14 }} \,\Omega \,{\text{cm}}^{ - 1}\), zijn soortelijk gewicht is \(1.03\,{\text{kg}}\ ,{\text{m}}^{ - 3}\) bij 25 °C heeft PDMS een betere thermische stabiliteit en is de thermische geleidbaarheid van PDMS \(0.15\,{\text{W}}\,{\text {m}}^{ - 1} \,{\text{K}}^{ - 1}\).[36] De glasovergangstemperatuur is slechts 125 °C, de thermische uitzettingscoëfficiënt (CTE) [37] is \(301\,{\text{ppm}}\,^{ \circ } {\text{C}} ^{ - 1}\). En de Young's modulus is \(\circa 3.7 \,{\text{MPa}}\) [38], waardoor de rek en rek meer dan 200% bedraagt. Gezien de uitstekende rekbaarheid, rekbaarheid, thermo-elektrische eigenschappen [39,40,41], hoge chemische stabiliteit en gebruiksgemak maken PDMS overvloediger in toepassingen zoals elektronische huid [42]. Polyimide (PI) materiaal met eigenschappen vergelijkbaar met PDMS (getoond in Tabel 1), PI vertoont een slechte thermische geleidbaarheid in de orde van \(0.1\,{\text{W}}\,{\text{m}}^{ - 1} \,{\text{K}}^{ - 1}\) [43,44,45], met elektrisch isolerende weerstand \({1}{\text{.5}} \times {10}^ {{{17}}} \, \Omega \,{\text{cm}}^{ - 1}\) [46]. De relatieve diëlektrische constante 3,0–3,6, met een hogere glasovergangstemperatuur (360–410 °C) [47] en een lagere thermische uitzettingscoëfficiënt (CTE) (\(16\,{\text{ppm}}\,^ { \circ } {\text{C}}^{ - 1}\)). Het is Young's modulus ≈\(2.8\,{\text{GPa}}\) [6, 48]. Polyurethaan (PU) materialen met biologische aanpasbaarheid, goede rekbaarheid [49], economisch en praktisch gebruik in temperatuursensoren voor bewaking van de menselijke lichaamstemperatuur [50]. Het dunne-film flexibele substraat heeft niet alleen uitstekende mechanische eigenschappen, maar is ook geschikt voor toepassingsonderzoek op het gebied van flexibele temperatuursensoren op basis van uitstekende thermische eigenschappen. Zoals getoond in Fig. 1. Naast het gebruik van de bovengenoemde organische polymeermaterialen in flexibele sensing, zijn gewone stoffen of andere biologisch afbreekbare materialen, zoals stof [51, 52], zijde [53] en katoen [54], ook zacht en vervormbaar, lichtgewicht, economisch, ademend, comfortabel, duurzaam en herbruikbaar. Andere voordelen, het wordt ook verwacht en betrokken om te worden bestudeerd als basismateriaal voor flexibele temperatuursensoren.
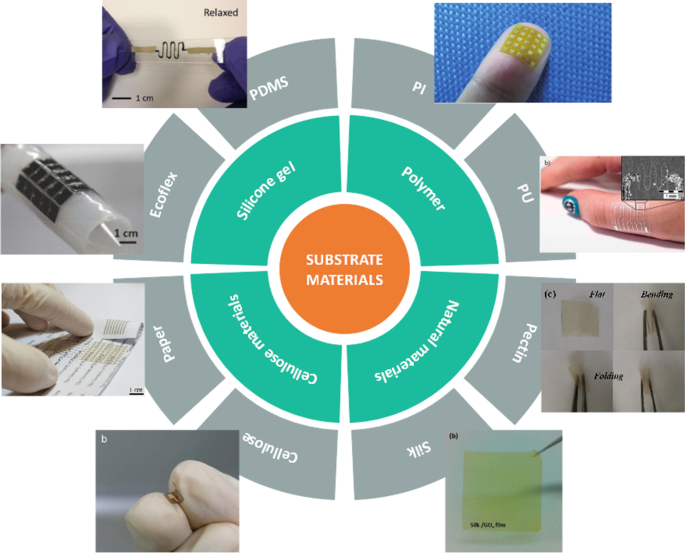
Schematische weergave van de substraatmaterialen voor een deel van flexibele sensoren. Met de klok mee van rechts boven:polyimide (PI) [55], polyurethaan (PU) [56], pectine [32], zijde [33], cellulose [57], papier [28], ecoflex [31] polydimethylsiloxaan (PDMS) [ 58]
Thermisch materiaal
Het actieve materiaal is gevoelig in de sensor die verantwoordelijk is voor het direct en effectief reageren op warmtebronnen en thermische signalen, de eigenschappen ervan bepalen direct de prestaties van de temperatuursensor, inclusief de gevoeligheid voor temperatuur, de lengte van de responstijd, de duurzaamheid en de resolutie tot temperatuur [59]. Een warmtegevoelig materiaal dat eenvoudig te fabriceren is, redelijk in grondstoffen, bio-aanpasbaar en een zekere mate van vervormbaarheid en uitstekende prestaties heeft, heeft meer aantrekkingskracht voor diepgaand onderzoek naar flexibele temperatuursensoren [60,61,62].
Koolstof
Er is veel moeite gedaan om temperatuurgevoelige geleidende composietmaterialen te maken door verschillende geleidende vulstoffen (zoals op koolstof gebaseerde materialen, geleidende polymeren en metaaldeeltjes) op te nemen in halfgeleiders en isolerende polymerenmatrices. Veelgebruikte koolstofmaterialen zijn onder meer carbon black (CB), grafiet (Gr), koolstofnanobuizen (CNT's) en grafeen.
Carbon black (CB) en grafiet worden vaak gebruikt als geleidende vulstoffen vanwege hun uitstekende elektronische en mechanische eigenschappen en lage verwerkingskosten. Onder hen is CB gemakkelijk om aggregaten te vormen wanneer het wordt gemengd met polymeren om composietmaterialen te vormen, en temperatuurveranderingen zullen de elektrische eigenschappen ervan beïnvloeden [63]. Stabiliteit resulteert in een hogere temperatuurweerstandscoëfficiënt (TCR) [64]. Grafiet is een allotroop van koolstof, met een goede elektrische geleidbaarheid, thermische geleidbaarheid en chemische stabiliteit, en een thermische uitzettingscoëfficiënt kleiner bij \(5.0 \times 10^{ - 6} \,{\text{K}}^{ - 1} \) [65]. Vergeleken met roet is grafietpoeder als geleidende vulstof gevoeliger voor temperatuur, en de twee en hun mengsels zullen de percolatiedrempel verlagen van de temperatuurgevoelige materiaalcomposietfilm gevormd door het gevulde polymeer. Geëxpandeerd grafiet (EG) is een nieuw type functioneel koolstofmateriaal dat losse en poreuze wormachtige substantie wordt verkregen uit natuurlijke grafietvlokken door intercalatie, wassen, drogen en expansie bij hoge temperatuur. De thermische geleidbaarheid van de composiet gevormd door heet persen nadat het materiaal \(4.70\,{\text{W}}\,{\text{m}}^{ - 1} \,{\text{K}} kan bereiken ^{ - 1} { }\) wanneer 10 gew.% EG wordt geïmpregneerd in PDMS-prepolymeer [66]. Shih et al. [67]gebruikte PDMS als het basismateriaal en grafietpoeder als het warmtegevoelige detectiemateriaal, en gebruikte de PI-film door het printproces een flexibele temperatuursensorarray samengesteld uit Gr/PDMS-composietsensoren (weergegeven in Fig. 2a). Vergeleken met de klassieke platina (Pt) dunne-film temperatuursensor (TCR = \({ }0.0055\,{\text{K}}^{ - 1}\)) in dezelfde meting van de soortelijke weerstand onder verandering van de omgevingstemperatuur (30–110 °C), wanneer de grafietvolumefractie 15% en 25% is, is de TCR van de composietmaterialen \(0.286\,{\text{K}}^{ - 1}\) en \ (0.042\,{\text{K}}^{ - 1}\), de resultaten bewijzen dat de gevoeligheid van het Gr-PDMS composietmateriaal hoger is. Huang en anderen hebben een met grafiet gevuld polyethyleenoxide (PEO) en polyvinylideenfluoride (PVDF) composietmateriaal [68], (Fig. 2b) onderzocht en vervaardigd dat gemakkelijk kan worden bevestigd aan het oppervlak van het menselijk lichaam met variabele kromming door een eenvoudig spincoatingproces. Een temperatuursensor met een detectiebereik van 25,0–42,0 °C, met een hoge resolutie van 0,1 °C en hoge cyclusstabiliteit, uitstekend anti-interferentievermogen (inclusief buigvast en waterdicht), en kan de sensor binnen 1 maand onderhouden. Het kan de temperatuur van de oksels langdurig continu meten. Zijn uitstekende mechanische eigenschappen zijn misschien omdat de Gr-deeltjes als vulstoffen in het composiet minder bewegen onder verschillende krommingen. De thermische prestatie is gerelateerd aan de thermische uitzetting van het polymeer. Als eendimensionaal nanomateriaal zijn koolstofnanobuisjes verbonden om een ruimtelijke topologische structuur te vormen [69]. De diameter is over het algemeen 2-20 nm. Het heeft veel abnormale mechanische eigenschappen (elastische modulus tot \(1 \,{\text{TPa}}\)), elektrische eigenschappen, waaronder elektrische geleidbaarheid tot \(10^{4 } \;{\text{S} }\,{\text{cm}}^{ - 1}\) [70], intrinsieke mobiliteit van dragers (\({10,000}\, {\text{cm}}^{2} \,{\text{V }}^{ - 1} \,{\text{S}}^{ - 1}\)) [71]. In de afgelopen jaren, met de verdieping van het onderzoek naar koolstofnanobuizen en nanomaterialen, zijn hun brede toepassingsmogelijkheden ook voortdurend onthuld. Koolstofnanobuisjes hebben uitstekende warmteoverdrachtprestaties en CNT's hebben een aanzienlijke aspectverhouding, dus ze zijn in de lengterichting. De prestaties van de warmtewisseling zijn zeer hoog en de toepassing van flexibele temperatuursensoren is ook voortdurend aan het innoveren, inclusief uitstekende ontwerpideeën en prestaties [72]. De composieten gevormd door koolstofnanobuizen en poly(3,4-ethyleendioxythiofeen)-poly(styreensulfonaat) (PEDOT:PSS) werden bestudeerd, en het bleek dat de prestaties van meerwandige koolstofnanobuizen (MWCNT's) en enkelwandige koolstof nanobuisjes (SWCNT's) waren niet hetzelfde [73]. Bij dezelfde temperatuur worden de composietmaterialen gevormd door MWCNT's en PEDOT:PSS later, terwijl de impedantie van de composiet wordt verlaagd, vermindert de composiet ook de gevoeligheid voor temperatuur en vochtigheid [74]. Kim et al. [75] gebruikte een natspinproces en gebruikte ook een PEDOT:PSS-composiet. De SWCNT's die ermee zijn samengesteld, kunnen de elektrische geleidbaarheid en arbeidsfactor van het composiet aanzienlijk verbeteren en de prestaties van het composiet verbeteren (Fig. 2d).
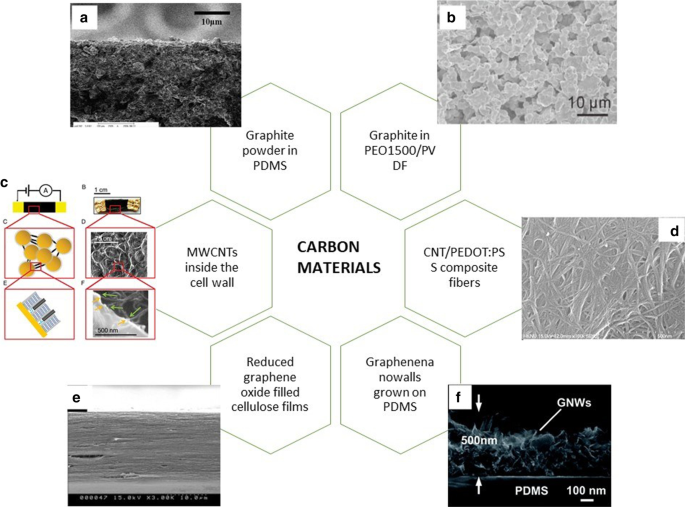
Diverse flexibele temperatuursensoren op basis van carbon materialen. een SEM-beelden van een grafiet-PDMS-composiet [67]. b De gehele oppervlaktemicrofoto van PEO1500/PVDF/Gr [68]. c Schematische diagrammen en scanning elektronenmicroscopie (SEM) beelden van cyberwood [76]. d SEM-afbeeldingen van de CNT/PEDOT:PSS-composietvezels met een CNT-gehalte van 40 gew.% [75]. e SEM-beelden van CrGO100 [54]. v Het dwarsdoorsnede-SEM-beeld van GNW's op PDMS [86]
Conventionele methoden voor het maken van biologische materialen zijn gebaseerd op reverse engineering van biologische structuren, bionica en biologische inspiratie. Biologische structuren presteren vaak beter dan door mensen gemaakte materialen. Hogere planten voelen bijvoorbeeld temperatuurveranderingen met een hoge responsiviteit. Getoond in Fig. 2c, Giacomo et al. [76] stelde een biomimetisch materiaal voor met een uitstekende temperatuurresponsgevoeligheid, gemaakt van geïsoleerde plantencellen en koolstofnanobuisjes (MWCNT) met behulp van plantaardige nano-biomimetische technologie. CNT's gebruiken als kanaal om cellen rechtstreeks met elkaar te verbinden, waardoor een bionisch materiaal ontstaat met een effectieve temperatuurweerstand (TCR) van \(- \,1730\% \,{\text{K}}^{ - 1}\), wat twee ordes van grootte hoger dan de huidige beste sensor, en het meettemperatuurbereik is 35–75 °C. Veel soorten onderzoek naar temperatuursensoren kunnen een hoge gevoeligheid bereiken in een smal temperatuurbereik, maar in een breed temperatuurbereik (40 K) zijn de responsprestaties onvoldoende. Zie ook de biologische temperatuurgevoelige structuur van slangen in het membraan van de natuur - slangenkuil, het biologische membraan heeft een uitzonderlijk hoge temperatuur- en afstandsgevoeligheid. Het kan worden gebruikt om warmbloedige prooien op een bepaalde afstand te lokaliseren. Ze gebruikten de toevoeging van Ca 2+ ionen aan de pectinefilm om het detectiemechanisme van de wangfilm van de slang na te bootsen om een temperatuurgevoelig pectinehydrogel-materiaal te bereiden waaraan CaCl2 is toegevoegd [10]. Het is mogelijk om temperatuurbronnen met een gevoeligheid van < 10 mK in kaart te brengen en te monitoren en warme objecten op een bepaalde afstand waar te nemen. Het combineren van plantencellen of het imiteren van dierlijke biologische structuren levert nieuwe ideeën op en maakt de onderzoeksrichting van flexibele sensoren breder.
Grafeen is een tweedimensionale hybride koolstoflaag met een hexagonaal honingraatrooster [77, 78]. Er is een groot aantal gedelokaliseerde elektronen, waardoor het unieke ladingstransport een hoge dragerconcentratie mogelijk maakt (\({10}^{{{33}}} \,{\text{cm}}^{ - 2}\)), onder bepaalde omgevingsomstandigheden is de mobiliteit groter dan \({10}^{5} \,{\text{cm}}^{2} \,{\text{V}}^{{ - {1}}} \, {\text{s}}^{ - 1}\). En de ruimtelijke structuur kan zorgen voor een overvloed aan bits dots. Functionele groepen worden aangepast om aan verschillende toepassingsvereisten te voldoen. Tegelijkertijd heeft het een hoge vloeibaarheid, uitstekende thermische geleidbaarheid, uitstekende transparantie, mechanische eigenschappen tot \(1 \,{\text{TPa}}\) elastische modulus, chemische stabiliteit en biocompatibiliteit [79]. Het heeft veel aandacht getrokken in de toepassingsgebieden van verschillende elektronische apparaten [80]. Transparant grafeenoxide (GO) of gereduceerd grafeenoxide (rGO) met uitstekende elektronische en mechanische eigenschappen is een product met een gelaagde structuur gevormd door grafietpoeder na oxidatie of verdere reductie van GO [81, 82]. Het oppervlak bevat hydroxylgroepen, carboxylgroepen en ringen. Veel functionele groepen, zoals oxygroepen, kunnen gemakkelijk worden gewijzigd en zijn gevoelig voor omgevingscondities, waaronder vochtigheid, temperatuur en chemische stoffen, en zichtbare responskenmerken [54, 83]. De lage geleidbaarheid van grafeenoxide (GO) is echter niet geschikt voor elektronische apparaten. Het gereduceerde grafeenoxide (rGO) wordt gesynthetiseerd door thermische reductie om de geleidbaarheid te verbeteren. Zijn uitstekende temperatuurgevoeligheid is ook vereist voor flexibele temperatuursensoren [54, 84, 85]. Grafeen nanowalls (GNW's) worden gekweekt tot grafeen nanosheets loodrecht op het substraat door gebruik te maken van plasma-enhanced chemical vapour deposition (PECVD) techniek [77] en polymeer-ondersteunde overdrachtsmethode. Het groeiproces vormt een verspringende structuur om het een hogere rekprestatie te geven [61, 83]. Uitstekende mechanische eigenschappen zijn ook toegepast op temperatuursensoren in onderzoek. Het gebruik van multifunctioneel grafeen en zijn derivaten in elektronische huidtoepassingen maakt de weg vrij voor flexibele temperatuursensoren met uitstekende prestaties, transparantie, rijke functies en een eenvoudig fabricageproces.
Yan et al. [58] heeft een flexibele temperatuursensor gefabriceerd die driedimensionaal geplooid grafeen als het actieve materiaal gebruikt en de temperatuur in het bereik van 30-100 ° C kan bewaken via een lithografische filtermethode [34]. Vanwege de bijzonderheid van de veerachtige structuur, kunnen de temperatuurveranderingsdetectiekenmerken worden gekarakteriseerd bij een rek tot 50%. De TCR (\(- \,1,05 \pm 0,28\% \,{\text{K}}^{ - 1}\)) van de sensor is onder ongespannen omstandigheden hoger dan de gerapporteerde silicium nanodraad temperatuursensor (\(0,15 - 0,37\% \,{\text{K}}^{ - 1}\)) keer. Het is vermeldenswaard dat de thermische index van het thermische materiaal van deze structuur kan worden aangepast. De temperatuurrespons en het herstel kunnen binnen tientallen seconden voltooid zijn, wat de toepasbaarheid vergroot in vergelijking met traditionele stijve keramische thermistoren. Liu et al. [87] gebruikte rGO-materiaal als het temperatuurgevoelige materiaal om een lichtgewicht en goedkope, flexibele temperatuursensor te fabriceren door middel van printtechnologie. De bewakingstemperatuur is 20-110 °C, de gevoeligheid is \(0.6345\% \,^{ \circ } {\text{C}}^{ - 1}\), en de reactietijd kan 1,2 s bedragen. Met specifieke spannings- en rekeigenschappen, kan worden bevestigd aan een specifiek krommingsoppervlak. Onder dezelfde experimentele omstandigheden, na vergelijking van gereduceerd grafeenoxide (rGO), enkelwandige koolstofnanobuizen (SWCNT's) en meerwandige koolstofnanobuizen (MWCNT's), bij de vergelijking van lineariteit, gevoeligheid, mechanische eigenschappen en herhaalbaarheid, wordt gevonden dat de prestaties van de temperatuursensor die rGO als het actieve materiaal gebruikt, het meest gebalanceerd is, wat ideeën oplevert voor de grootschalige voorbereiding van elektronische huid in de toekomst. Sadasivuni et al. [54] stelde een composietfilm voor met cellulose als matrix en thermisch rGO als vulmiddel om een flexibel en efficiënt temperatuurbereik van 25-80 °C te produceren, afhankelijk van temperatuurveranderingen. (getoond in Fig. 2e) Capacitieve flexibele temperatuursensor met een lineair verband. Vergeleken met de standaard commerciële platina-temperatuursensor, zal de temperatuursensor in de loop van de tijd geen vervuiling veroorzaken door metaalcorrosie en kan de stabiliteit lange tijd behouden blijven. Trung et al. [17] fabriceerde een transparante en rekbare (TS) flexibele temperatuursensor door middel van een eenvoudige spincoatingmethode en lamineertechnologie. De temperatuurgevoelige laag gevormd door rGO nanosheets en een elastisch polyurethaan (PU) substraat. Samengestelde materiaalvorming. De nieuwigheid van het elektronische apparaat is dat elke laag materiaal in de structuur TS is en gemakkelijk direct kan worden gecoat op een transparant en rekbaar substraat. Het kan dan gemakkelijk aan het menselijk lichaam worden bevestigd. Het apparaat kan temperatuurveranderingen tot 0,2 °C detecteren. Na 10.000 keer uitgerekt te zijn met een belasting van 30%, heeft het bijna geen effect op de temperatuurrespons. Het apparaat kan nog steeds worden gebruikt als de belasting 70% is. Yang et al. [86] stelde voor om plasma-enhanced chemical vapour deposition (PECVD)-technologie te gebruiken om een speciale geïnterlinieerde 3D geleidende GNW-netwerkstructuur op koperfolie te laten groeien (Fig. 2f), gecombineerd met een polymeer-ondersteunde overdrachtsmethode en PDMS om een ultragevoelige draagbare temperatuursensor. De thermische respons is veel groter dan die van traditionele temperatuursensoren. De uitstekende ductiliteit en thermische gevoeligheid van GNW's in combinatie met de grote uitzettingscoëfficiënt van PDMS stellen de sensor in staat om de temperatuurverandering van 25–120 °C te bewaken met een nauwkeurigheid van 0,1 °C, een responstijd van 1,6 s en een hersteltijd van 8,52 s , en binnen enkele maanden stabiel te houden. TCR bereikt \(0.214\,^{ \circ } {\text{C}}^{ - 1}\), wat twee ordes van grootte hoger is dan de standaard commerciële platina temperatuursensor (\(39,2 \times 10^{ - 4 } \,^{ \circ } {\text{C}}^{ - 1}\)). Het gebruik van koolstofmaterialen in flexibele temperatuursensoren heeft het toepassingspotentieel in gezondheidsmonitoring, draagbare apparaten, robotica, mens-machine-interfaces en kunstmatige huid bevorderd.
Metaal en metaaloxide
Metalen materialen zijn over het algemeen geleidende materialen zoals goud (Au) [88,89,90], zilver (Ag) [91, 92], koper (Cu) [93] en platina (Pt) [55, 94] (in Fig. 3c), nikkel (Ni) [95] en aluminium (Al) [96], die voornamelijk werden gebruikt als elektroden en draden van sensoren. Vergeleken met de traditionele stijve metalen temperatuursensor, heeft de flexibele metalen temperatuursensor een hoge mechanische flexibiliteit, kan gemakkelijk worden bevestigd aan sterk gebogen oppervlakken en is meer geschikt voor het detecteren van kleine temperatuurveranderingen en verdelingen in een klein bereik. Wat het huidige drukproces betreft, zijn sommige metalen materialen temperatuurgevoelig en goed geleidend, en zijn ze gemaakt van geleidende metalen nano-inkt [97], nanovulmiddel [95], nanodraad [98] en film met patroon om een actieve temperatuurgevoelige laag [19] te maken, die veel wordt gebruikt in flexibele temperatuursensoren.
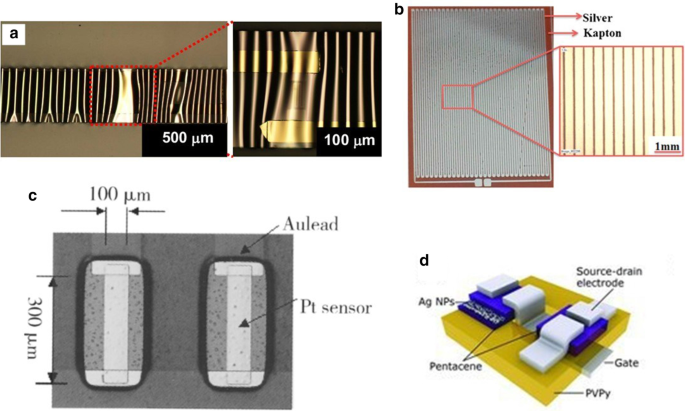
Diverse flexibele temperatuursensoren op basis van metalen materialen. een Rekbare sensoren bovenop een PDMS-substraat met periodiek geknikte patronen [99]. b Foto's van inkjet geprinte zilveren temperatuursensor op Kapton-substraat [102]. c Een afbeelding van de temperatuursensoren [6]. d De schematische diagrammen van de (één transistor)–(één thermistor) temperatuursensor [101]
De meest voorkomende gevoelige materialen in typische temperatuursensoren zijn Pt en Au. Bin et al. [6] gebruikte de Micro-Electro-Mechanical System (MEMS) -technologie en stelde platina voor als het detectiemateriaal dat na een pelproces op de PI-film werd afgezet. Nadat het lamineren het vereiste patroon heeft gevormd, wordt het gebruikt om de temperatuurverandering van 20-120 °C te bewaken, en de TCR-waarde is \(0.0032\,^{ \circ } {\text{C}}^{ - 1}\ ). Vergeleken met het dure platina heeft Au een betere geleidbaarheid en flexibiliteit. Om te realiseren dat de prestaties van het apparaat niet kunnen worden beïnvloed door trekspanning, Yu et al. [99] slim gebruikte sputterafzetting op een voorgerekt PDMS flexibel substraat Chromium(Cr)/Au dunne film (in figuur 3a), en een omkeerbare buigbare en rekbare flexibele temperatuursensor wordt gefabriceerd door middel van een fotolithografieproces. Wanneer de maximale rek van het apparaat 30% is, zullen de prestaties van het apparaat niet veranderen. Het onderzoek verbetert het defect van een slechte trekweerstand van flexibele sensoren. Dankoco et al. [100] gebruikte een organische zilvercomposietinkt om zilverlijnen op een polyimidefilm glad en gelijkmatig af te zetten door middel van inkjetprinten (zie in figuur 3b). Het maakte een meetbare lichaamsoppervlaktetemperatuur van 20-60 ° C kan een gebogen en flexibele temperatuursensor zijn. De gemiddelde gevoeligheid is \(2.23 \times 10^{3} \,^{ \circ } {\text{C}}^{ - 1}\). De sensor heeft echter < 5% hysterese. Ren et al. [101] stelde een flexibele temperatuursensor voor met een hoge thermische resolutie (dynamisch bereik = 10 bits) op basis van de integratie van zilveren nanodeeltjes (NP's)/pentaceenthermistor en organische dunnefilmtransistor (OTFT) met een temperatuurbereik van 15-70 ° C. Het onderzoek testte de hoge afhankelijkheid van composietmaterialen van temperatuur en bewees de haalbaarheid van zilveren nanodeeltjes (NP's) in thermistortoepassingen. Sensoren met een hoog dynamisch bereik zijn ook geschikt voor sensorarrays met een groot oppervlak en elektronische huid. Jeon et al. [95] ontwikkelde een flexibele temperatuursensor ontworpen met een mengsel van semi-kristallijne polyethyleen (PE) en polyethyleenoxide (PEO) polymeren gevuld met nikkeldeeltjes. Onder hen, wanneer de concentratie van nikkeldeeltjes de permeatiedrempel van nikkelvulstoffen overschrijdt, kan een hoge geleidbaarheid (\(40 \,{\text{S}}\,{\text{cm}}^{ - 1}\)) worden verkregen. Met de grote positieve temperatuurcoëfficiënt (PTC) die uniek is voor het binaire polymeermengsel, kan de gefabriceerde sensor niet alleen een instelbaar gevoelig temperatuurbereik bieden, terwijl de stabiliteit van de thermische cyclus behouden blijft om een herhaalbaar temperatuurresponsproces te bereiken. De sensor heeft drie ordes van grootte hogere gevoeligheid (\(0.3\, {\text{V}}\,^{ \circ } {\text{C}}^{ - 1}\)) dan standaard thermokoppels en draadloze transmissie functie, maar er is een significante fout van ± 3.1 °C. Het is vermeldenswaard dat ze draadloze detectietechnologie willen combineren met afdrukbare materialen om een breed scala aan toepassingen te bereiken.
Metaaloxide is ook een belangrijk actief materiaal en wordt veel gebruikt in temperatuursensoren. De hoge temperatuurbestendigheidscoëfficiënt van metaaloxidematerialen kan de temperatuurwaarnemingsprestaties verbeteren. De thermische gevoeligheid van metaaloxidehalfgeleiders is een fenomeen waarbij de halfgeleiderverbinding verandert bij verschillende temperaturen en de weerstandswaarde verandert. Liao et al. [103] meldde een zeer gevoelige temperatuur/mechanische sensor met twee parameters die anorganisch thermisch materiaal vanadiumdioxide bevat (VO2 ), op basis van PET/vanadiumdioxide vervaardigd door middel van transferprinttechnologie (VO2 )/PDMS meerlagige structuur. De VO2 laag afgezet door polymeer-assisted deposition (PAD) technologie wordt geëtst en bevestigd aan de PDMS-film. Het kan de temperatuur detecteren in het bereik van 270-320 K. De temperatuurwaarnemingsprestaties met een resolutie van 0,1 K toegeschreven aan de hoge TCR van de VO2 materiaal. Om veranderingen in lichaamstemperatuur nauwkeurig in kaart te brengen, hebben Huang et al. [91] ontwikkelde een inkjetprintproces. Nikkeloxide (NiO) gebruiken om een stabiele nanodeeltjesinkt op de PI-film te genereren. De kleine vierkante NiO-film wordt aan de uiteinden tussen de zilveren geleidende sporen gedrukt om snel temperatuursensoren te fabriceren. Het kan nog steeds presteren onder de buigtest en heeft een vergelijkbare reactiesnelheid als een thermokoppel. Het uitgebreide onderzoek naar metaal en metaaloxide heeft de basis gelegd voor de toepassing van metalen materialen op het gebied van flexibele temperatuursensoren op basis van de thermische eigenschappen van metalen materialen en heeft interessante verkenningen opgeleverd.
Polymeren en organische materialen
Polymeren zijn de meest gebruikte materialen in flexibele sensoren. Naast dat ze worden gebruikt als substraten of actieve middelen, zijn thermisch gevoelige composietmaterialen met mechanische flexibiliteit, lichtgewicht, transparantie, stabiele prestaties, gemakkelijke verwerking en lage fabricagekosten flexibel. De toepassing in de temperatuursensor heeft veel aandacht getrokken. Thermogevoelige polymeren die vaak in temperatuursensoren worden gebruikt, zijn onder meer poly(3,4-ethyleendioxythiofeen)-poly(styreensulfonaat) (PEDOT:PSS) [16, 104,105,106], poly(3-hexylthiofeen) (P3HT) [107], polypyrrool ( PPy) [57], pentaceen [101], poly (N-isopropylacrylamide) (pNIPAM) [108], poly(vinylideenfluoride) (PVDF) [109.110.111.112.113], enz.
Based on a circuit design strategy [114] that can improve the accuracy and robustness of a stretchable carbon nanotube temperature sensor, Zhu et al. [115] used differential readout technology to compare the composition of the active, sensitive layer of a stretchable temperature sensor based on OTFTs (see Fig. 4a). Among them, Polystyrene- block-poly(ethylene-ran-butylene)-block-polystyrene (SEBS) with azide-crosslinke and Poly(diketopyrrolopyrrole-[3,2-b]thieno[2′,3′:4,5]thieno[2,3-d]thiophene]) (PDPPFT4) and Poly(isoindigo-bithiophene) (PII2T) these two organic semiconductors (OSCs) are blended and spin-coated on the gate dielectric, CNTs are used as electrodes, and the temperature measurement range is 25–55 °C. Inside, the temperature coefficients of the two sensors are \(- \,2.89\% \,^{ \circ } {\text{C}}^{ - 1}\) and \(- \,4.23\% \,^{ \circ } {\text{C}}^{ - 1}\), respectively. When the uniaxial strain range is 0–30%, the errors are < 1 °C and < 1.5 °C, respectively, further show the feasibility and generalizability of differential readout method and OSCs in stretchable sensors are discussed. Yokota et al. [116] reported a large-area super-flexible temperature sensor based on a semi-crystalline acrylate polymer/graphite composite material that can be measured at multiple points and can be printed (in Fig. 4b). Between 25 °C and 50 °C, it shows noticeable resistance changes at this temperature, which is suitable for measuring the physiological temperature changes of the human body. It has stable thermal cycle stability, the sensitivity of up to 20 mK, and a high-speed response time of less than 100 ms. In in vivo experiments, the stable changes in the rat's lungs' core temperature measured, but the high resolution of the sensor proved to be 0.1 °C. The sensor array based on the above characteristics realizes the dynamic visual, thermal imaging demonstration of the spatial temperature change. However, the air permeability of the equipment components is not right, and the long-term wear is one of the problems to be solved.
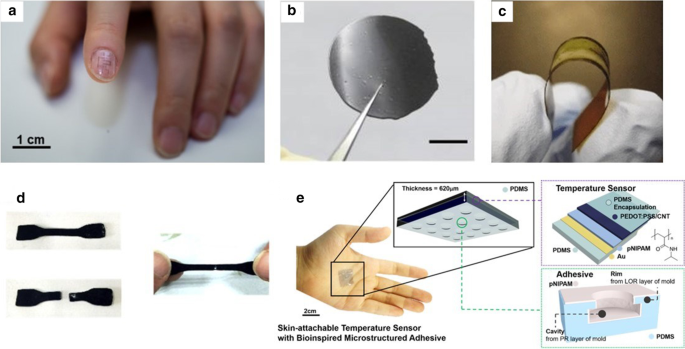
Various flexible temperature sensors based on thermosensitive polymer. een A sample with two temperature sensors on a fingernail [115]. b Photograph of a film of copolymer with graphite filler (scale bar, 1 cm) [116]. c Photograph of a Te-nanowire/P3HT-polymer composite device on a flexible Kapton substrate [107]. d Photos of the DN hydrogels self-healing process [121]. e Photographic image of the fabricated temperature sensor attached onto palm skin and the overall schematic illustration of the octopus-mimicking microstructured adhesive [108]
Self-supplied energy has always been the focus of many people's attention [117]. The realization of self-supplied energy by flexible equipment will significantly reduce the equipment's need for external energy, making flexible equipment more portable and more straightforward [118]. Among them, the thermoelectric polymer materials are the realization of self-supplied energy required. Yang et al. [107]developed a flexible thermoelectric nanogenerator (TENG) based on a clean composite thermoelectric material formed by Te-nanowires grown at room temperature and poly(3-hexyl thiophene) (P3HT) polymer (shown in Fig. 4c). TENG can generate electricity only with a temperature difference of 55 K. Because of the characteristics of thermoelectric materials, TENG can be used as a flexible temperature sensor to monitor the temperature difference of the entire device, and use human body temperature as an energy source to directly power the sensor. The monitoring sensitivity at room temperature is 0.15 K. Besides, they also demonstrated another self-powered temperature sensor with a response time of 0.9 s and a minimum temperature change of 0.4 K at room temperature. The small temperature resolution makes the sensor device can monitor the temperature change of the fingertip. Self-supplied can make flexible sensor equipment more independent and reduce weight. It is also a possibility for the development of flexible sensors in the future (Table 2).
Low-cost, environmentally friendly, easy-to-obtain, and process materials with excellent biocompatibility have always been an essential condition for human beings to pursue to meet mass production continuously [119]. There is such an almost inexhaustible biological material–cellulose, which has excellent properties. Its elasticity and other advantages also play an essential role in flexible sensor devices and can be used as a flexible substrate. Polypyrrole (PPy) is a linear biocompatible polymer with excellent electrochemical stability and rapid response. Mahadeva et al. [57] reported a method based on in-situ polymerization-induced adsorption that combines unique cellulose and nano-thickness polypyrrole (PPy) excellent electrical properties to form a temperature and humidity sensitive composite. The material used to fabricate an environmentally friendly, low-cost, bio-adaptable flexible temperature sensor. Because of the sensitivity of materials to humidity, as the temperature increases, the sensor's capacitance also increases. Hydrogels have received continuous attention in recent years of research [120, 121]. Because of its good self-healing ability, excellent toughness and stretchability, and biological adaptability, it has aroused great research interest in application fields such as flexible electronics, health monitoring, and biomedical diagnosis [122, 123]. However, ionic hydrogel, as a good ion conductor, can respond to a variety of stimuli, hydrogels with weak mechanical strength, and reduced temperature sensitivity present challenges in applying flexible temperature sensors. To solve the disadvantages of traditional hydrogels, An et al. [121] proposed a double-mesh ion-conducting double-network (DN) hydrogel with excellent temperature sensors' self-healing properties. The DN hydrogels self-healing process is shown in Fig. 4d. The addition of carbon nanotubes with high thermal conductivity to the hydrogel with dynamic physical crosslinking and high conductivity hydrophobic association network and ion association network improves the temperature sensitivity of DN hydrogel. The linear hydrogel temperature sensor can perfectly fit the surface of complex objects and produce sensitive resistance changes. The research and development of this material expand hydrogels' application in the fields of biomedicine and flexible electronics.
In recent research, PEDOT:PSS is a new type of organic conductive polymer that often used in printable, flexible temperature sensors [124]. Generally speaking, PEDOT:PSS has the advantages of high conductivity (\(10^{3} \,{\text{S}}\,{\text{cm}}^{ - 1}\)), excellent thermoelectric performance [125,126,127,128], strong stability [123, 129], and transparency when doped [60]. Most polymers are p-type semiconductors. By adding some solvents, such as dimethyl sulfoxide(DMSO) [130] or polyhydroxy organic compounds, such as ethylene glycol [131], the conductivity rate of the polymer can be increased dozens of times or even hundreds of times. Harada and colleagues used different composite ratios of CNTs and conductive PEDOT:PSS solutions to produce a series of composite heat-sensitive films with temperature sensitivity within \(0.25 - 0.78\% \,^{ \circ } {\text{C}}^{ - 1}\) through various printing processes [11, 132,133,134]. The sensing performance is better than the typical metal temperature sensor. Some of the devices exhibited a near body temperature resolution of less than 0.1 °C or fast response time of 90 ms. The research team used various printing methods to create a variety of flexible temperature sensors with different structures and outstanding performance, in addition to enriching the flexible temperature sensors applications in medical and health, wearable devices. Have also triggered everyone thinking about printable, flexible sensors. The fabricating details of printable, flexible temperature sensors will be expanded in the next unit. In addition to changing the composite ratio will affect the performance of the composite film, the structural improvement will also optimize the properties of the same composite film. As Fig. 4e shown, Oh et al. [108]demonstrated a biological material made by a photolithographic stripping process and a spin coating process, inspiringly imitating the adhesion structure of octopus foot sucker. It is a high-sensitivity resistor temperature sensor composed of poly(n-isopropyl acrylamide) (pNIPAM) temperature-sensitive hydrogel, PEDOT:PSS and CNTs. The device has a sensitivity of \(2.6\% \,^{ \circ } {\text{C}}^{ - 1}\) in the temperature range of 25–40 °C, and can accurately detect skin temperature changes of 0.5 °C. Because of the microstructure similar to the suction cup and its viscosity, the device has a certain degree of resistance to bending, non-irritating, long-lasting, and reusable binding effect.
Transparent and scalable nanocomposite field-effect transistor based on polyvinylidene fluoride (PVDF) and its copolymer polyvinylidene fluoride (P (VDF-TrFE)) with high stability, strong mechanical properties, and low distortion, it is often used in pressure sensing, strain sensing, and infrared (IR) light-sensing devices [135, 136]. Interestingly, researchers have found that this type of device is also highly responsive to infrared radiation from the human body, so it is predicted to be used to monitor the human body's physiological temperature changes [137]. Trung and colleagues, based on previous research experience [113, 138], use rGO/(P(VDF-TrFE)) composite sensing active layer as the channel through a simple spin coating process, integrated PEDOT:PSS can fabricate adjustable flexible field-effect transistor (FET) temperature sensor [139]. The film's temperature response and transparency can adjust by changing the concentration of rGO and the thickness of the composite film (Fig. 5a). The sensor can monitor temperature changes from 30 to 80 °C. With 0.1 °C resolution and monitoring ability, and super high-temperature response, excellent temperature sensing performance verifies the feasibility of the application of pyroelectric polymer materials in the field of softcore temperature sensors. Similarly, Tien et al. [112] in order to realize that the sensor can collect pressure and temperature signals without mutual interference, they proposed the use of field-effect transistor (FET) sensing platform to change the material of the response sensitive layer, based on their previous the research concluded that a mixture of polyvinylidene fluoride (P(VDF-TrFE)) and BaTiO3 (BT) nanoparticles (NPs) is used as piezoelectric (see Fig. 5b), pyroelectric gate dielectric and pentacene are used as organic semiconductor channel for pressure thermal resistance directly integrated into the FET platform when the flexible sensor is under multiple stimuli, it decouples the output signal and minimizes the signal interference of strain coupling. The FET sensor can disproportionately present strain and temperature at the same time. The FET sensor array can also visually respond to stimuli, exhibiting the advantages of low energy consumption and low failure, which shows a possibility for the application of large-area multi-modal flexible sensors in the field of electronic skin in the future. Flexible multi-parameter OFET devices that can be printed and fabricated in a large area have excellent application potential in biomedical monitoring, infrared imaging, and electronic skin.
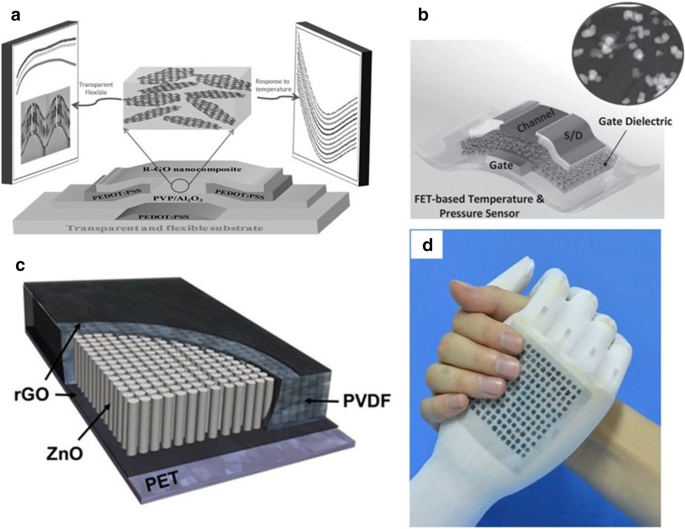
Flexible temperature sensor containing PVDF material. een Schematic of transparent, flexible rGO/P(VDF-TrFE) nanocomposite FET. The schematic illustrates structural, optical (transparent) and electrical (response to temperature) properties of the transparent, flexible R-GO/P(VDF-TrFE) nanocomposite FET [113]. b The structure of physically responsive field-effect transistor (physi-FET) with the bottom-gated and top-contact structure, where the gate dielectric is comprised of nanocomposite of P(VDF-TrFE) and BaTiO3 nanoparticles and the channel is organic semiconductor of pentacene [112]. c Schematic diagram of the ZnO/PVDF composite film and rGO electrodes [110]. d Photograph of the flexible MFSOTE matrixes [23]
In addition to the decoupling method to reduce or eliminate signal interference, in order to solve the problem of mutual interference of multi-parameter flexible sensor signals, Lee et al. [110] proposed a method of inferring temperature based on the recovery time of the resistance change signal, so that the semiconductor zinc oxide (ZnO) nanostructure is mixed into the substrate polyvinylidene fluoride (PVDF) as a filler (As shown in Fig. 5c) to make a highly sensitive multifunctional sensitive layer that can collect temperature and pressure signals at the same time. Among them, the semiconductor ZnO can increase the dielectric constant of PVDF, and it also has thermal stability. Zhang et al. [23] reported a dual-parameter flexible sensor based on a self-powered microstructure-frame-supported organic thermoelectric (MFSOTE) material. The Fig. 5e show the flexible MFSOTE matrixes. By converting the signal changes caused by temperature and pressure stimulation into two independent electrical signals, the temperature and pressure simultaneously sensed. This unique material shows excellent temperature sensing characteristics. The monitoring temperature range is 25–75 °C, The resolution can achieve < 0.1 K, the response time under 1 K temperature difference is < 2 s, and it can also be adjusted according to different substrates to meet the sensing needs.
Fabrication
With the increasing requirements for flexibility, multi-function, simple fabricating, and high sensitivity of electronic devices, the exploration and discovery of flexible sensor fabricating methods with a lightweight, simple process, low cost, and large-area fabricating have always been what researchers are keen [140]. This section mainly summarizes the recently reported and feasible fabricating strategies of flexible temperature sensing elements and discusses the key processes to improve their performance.
Thin Film Deposition
The thin film preparation method can be divided into vapor deposition and phase deposition according to the phase of the material used. The phase deposition includes spin-coating and inkjet printing processes mentioned later. In contrast, the vapor deposition depends on whether the deposition process contains the chemical reaction process divided into physical vapor deposition (PVD) and chemical vapor deposition (CVD).
PVD is to depositions or atoms generated by physical methods on a substrate under vacuum conditions to form a thin film, which generally used to prepare electrodes or active metal layers [141, 142]. Common deposition methods include vacuum evaporation, vacuum sputtering, and ion plating. Among them, the metal target ion sputtering refers to the vacuum container, under the action of high voltage 1500 V, the remaining gas molecules are ionized to form plasma, and the cations bombard the metal target under the acceleration of the electric field, causing the metal atoms to sputter on the surface of the sample to form conductive film [143]. Ahmed et al. [144] introduced a Si-temperature sensor based on a flexible PI substrate. They deposited undoped amorphous silicon as a sensing material between metal electrodes formed by radio frequency magnetron sputtering and packaged them. Finally, the temperature sensing element is embedded in the flexible polyimide film, and the sensing performance is not affected. The maximum TCR at 30 °C is \(0.0288\,{\text{K}}^{ - 1}\). Webb et al. [145] introduced two ultra-thin, skin-like sensor fabricating methods that are self-assembled on the skin surface in the form of an array to provide clear and accurate thermal performance monitoring. A structure is composed of a temperature sensor array, the sensitive layer formed by the serpentine trace structure of the Cr/Au layer deposited on the PI film by the metal evaporation deposition method, the microlithography technology, and the wet etching technology, and the reactive ion etching and metal deposition for contacts and interconnections complete the array. Another sensor structure uses multiplexed addressing to form a patterned PIN diode sensor design of doped Si nano-film. The sensitivity layer is defined by metal evaporation, photolithography, chemical vapor deposition, and wet etching steps. The two arrays are shown in Fig. 6a. Aluminum phthalocyanine chloride (AlPcCl) is often used as a material for solar cells and humidity sensors. Under the study development of Chani et al. [146] AlPcCl is used as a thermistor and deposited on an aluminum electrode on a glass substrate using a vacuum thermal evaporator. The authors found that the AlPcCl film has a higher sensitivity to the temperature at 25–80 °C, and annealing can improve sensing performance. In a flexible temperature sensor developed by Bin et al. [6] that uses Pt resistors as the thermosensitive material, Pt is evaporated on the Al layer deposited on the spin-coated PI film, and the Pt layer is patterned as a sensitive the layer is spin-coated and packaged with polyimide material. After hydrochloric acid treatment, a complete flexible temperature sensor is peeled off, which can be used to measure the surface temperature of objects in the biomedical field.
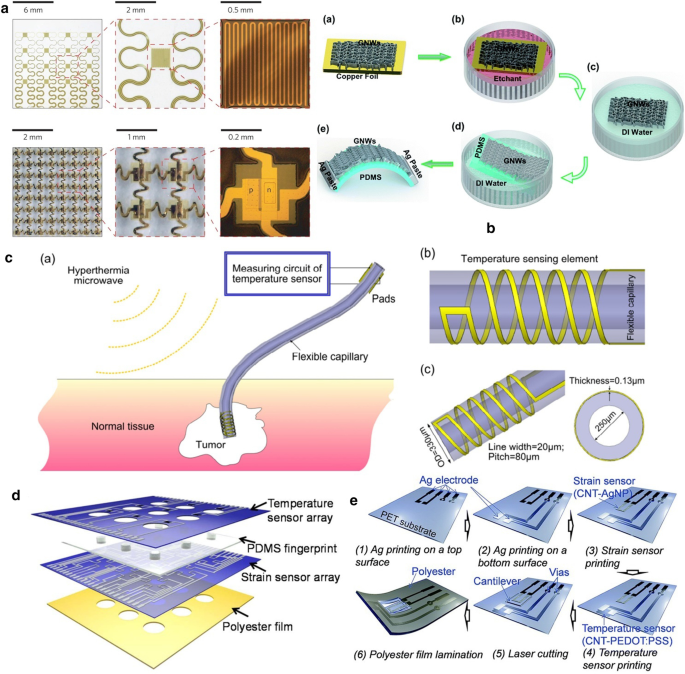
Fabrication method of flexible temperature sensor method of flexible temperature sensor. een Top:Optical images of a 4 × 4 TCR sensor array integrated on a thin elastomeric substrate with magnified views of a single sensor. Bottom:Optical images of a 8 × 8 Si nanomembrane diode sensor array integrated on a thin elastomeric substrate with magnified views of a single sensor [145]. b Schematic process for the fabrication of GNWs/PDMS temperature sensors [86]. c Sketch of the implantable micro temperature sensor on polymer capillary and its application. The head spiral sensing element is fabricated by photolithography [157]. d Schematic for each layer of the e-skin device [132]. e Schematic of the e-skin fabrication process on a PET substrate using a printing method [134]
Compared with other thin-film preparation processes, the chemical vapor deposition method can achieve high-purity and high-quality thin films. It can be structured and controlled at the atomic layer or nanometer level [147,148,149]. The process of synthesizing GNWs film on copper foil by low-pressure radio frequency plasma enhanced chemical vapor deposition (RF-PECVD) technology. Yang et al. [86] developed a flexible temperature sensor based on GNWs/PDMS. The fabricating process is shown in Fig. 6b. They verified GNWs is feasible as an active layer of a temperature sensor, and its thermal response performance exceeds that of a traditional metal temperature sensor. Compared with traditional CVD technology, using PECVD technology [150] under low temperature and low-pressure conditions can effectively improve the deposition rate and film quality. In another study, Zhou et al. used the floating catalyst chemical vapor deposition (FCCVD) method [151] to synthesize the original SWCNT film with a controllable thickness directly. The continuous network of CNTs grown by this method has significant conductivity and a high favorable Seebeck coefficient. After transferring the original SWCNT film to the PET substrate, drop-cast the branched polyetherimide (PEI) ethanol solution, and dry it to obtain an n-type SWCNT film that can be used in the fabricate of flexible thermoelectric modules. Although CVD can achieve the deposition of any material on any substrate, as the demand for simple, low-cost and large-area fabricating nanodevice fabricating technology continues to grow, the fabricating process is complex, high-cost, and toxic CVD growth processes and The time-consuming etching process is being replaced by more suitable flexible electronic device fabricating technology [58].
Micro-nano Patterned Fabrication
Thin-film patterning is one of the core technologies of flexible electronics fabricating. It follows the basic idea of removing materials from top to bottom or adding materials from bottom to top in the fabricating industry. Its key technologies are thin-film fabricating, patterning, transfer, replication, fidelity, and other crafts. Flexible electronics require large-area, low-temperature, low-cost patterning technology. Learned from the patterning technology of microelectronics and micro-electromechanical devices. However, at the same time, we must consider the characteristics of flexible electronic devices such as flexible substrates, organic materials, and large areas. The patterning technologies currently available for flexible temperature sensors include lithography, printing, soft etching, nanoimprinting, inkjet printing, laser sintering, transfer printing, nano-direct writing [152], and other processes.
Lithography is a patterning method to realize various and ingenious geometric figures or structures in flexible electronics. The photolithography process involves transferring the pattern on the photomask to the substrate by using the photoresist with different sensitivity and physical and chemical reactions under the light. The photolithography process usually uses photoresist on an insulator (usually a silicon wafer) to pattern the required pattern or structure after spin coating, and further realize it through a stripping process [153, 154]. Because of the photolithography process and the stripping process, high alignment and etching accuracy, simple mask production, and comfortable process conditions can usually achieve high-precision, feature-rich microstructure systems. In the ultra-thin flexible suture application with an integrated temperature sensor and thermal actuator developed by Kim et al. [155], they used photolithography technology to micro-process the equipment, and the fabricated flexible medical equipment has the stable thermal performance. Lithography technology limited by the necessity of available materials and precision equipment. The thickness of processable materials and thin films are limited. It is not suitable for device fabricating processes that require a large number of active materials. Yang et al. [156] proposed a flexible implantable micro temperature sensor, and used surface microlithography to etch the micro flexible temperature sensor on the outer surface of the polymer capillary (The sensing principle diagram of the miniature thermometer is shown in Fig. 6c). Using Pt as a sensitive material has good linearity, and it has a promising future as an implantable temperature sensor in the biomedical field. However, this technology is the foundation of the microelectronics industry and pioneered the era of wearables flexible electronics.
With the development of science and technology, the printing process has expanded from the traditional text and image field to the micro-nano structure patterning field. technology can deposit various materials on various substrates, and the printing process is not harsh on the environment. In a nutshell, printing technology includes letterpress, lithography, gravure, screen printing, and has evolved into soft etching [158, 159], transfer printing, nanoimprinting, and other methods. According to the specific implementation method, the wearable sensor can distinguish the printed part from the non-printed part with the mask help. In mask printing, the pattern to be transferred must be designed in advance and then formed through the mask. The functional, active material can directly be transferred to the substrate or electrode through the functional ink imprinting process [160]. Screen printing is a typical mask printing technique [161]. In the printing process by absolute pressure, the functional ink is transferred to the substrate through a squeegee with a patterned mesh to form a pattern. The unique printing method allows screen printing to achieve fast, large-area low-cost fabricating requirements on flat or curved surfaces. It has been widely used in fabricating sensor working circuits, electrodes, and sensor sensing elements. Compared with photolithography technology, screen printing can produce patterns on various materials. However, its pattern resolution cannot meet complex geometric shapes requirements and is only suitable for making patterns with simple shapes. Yokota et al. [116] stirred and mixed a variety of semi-crystalline polymers with graphite to form a super-flexible temperature-sensitive copolymer for flexible temperature sensors for human physiological temperature monitoring. The super-flexible temperature sensor element is printed by mask printing by sandwiching the copolymer mixed with graphite filler between two interdigital gold electrodes deposited on the PI film and then forming by hot pressing. Yan et al. [58] used a flat-plate suction filter printing method to deposit graphene through a mask, vacuum filter it, and transfer it to the substrate to form a three-dimensional fold pattern structure produce stretchable graphene with a variable thermal index. The thermistor increases the sensing area and stretchability of the sensor. The pre-designed stretchable sensitive material pattern can still maintain sensitive monitoring of temperature when stretched to less than 50%. To achieve the economical fabricating of sensors with a larger area, Harada and colleagues abandoned complex and costly fabricating processes (such as deposition and photolithography). They chose to fabricate a series of multifunctional flexible sensors using only printing processes. The PEDOT:PSS/CNT composite ink printed on the circuit formed by screen printing on the PET substrate through a shadow mask, and there are holes after laser writing (LS) to combine with the lower layer of PDMS. The fingerprint-like structure (see in Fig. 6d) is combined with the screen-printed strain sensor layer to form a flexible sensor array. The deformation and temperature difference caused by the contact contacts achieve a human-like monitoring performance. In another study, also using full printing technology, Kanao et al. [134] proposed a multifunctional flexible sensor array based on a cantilever beam structure (Fig. 6e). They placed strain sensors and temperature sensors on a flexible screen-printed circuit. On both sides of the PET substrate, a patterned shadow mask with a flexible temperature sensor (PEDOT:PSS/CNT composite ink) printed on the screen's electrical contacts printed circuit. The fully printed array sensor used to imitate the sensing characteristics of human skin. When the cantilever beam structure strained, the heat source is closer to the temperature sensor on the substrate's bottom surface to monitor temperature changes more accurately.
Transfer printing is a printing method that the patterned surface concave structure or convex structure transferred to the receptor substrate through a non-patterned stamp. The basic principle is to use the different viscosity of the printing layer relative to the stamp and the substrate to achieve pattern transfer [162,163,164,165]. There are two types of transfer printing:direct transfer printing and indirect transfer printing. In the fabricate of flexible temperature sensors, the latter often used, that is, the use of a pre-printed patterned film to transfer to the receptor substrate. In the previous review, many examples of organic materials are sensitive layers mentioned, but few inorganic materials are used as temperature-sensitive materials. It is worth noting that Liao et al. [103] reported a high-sensitivity temperature/mechanical dual-parameter sensor containing inorganic thermal material vanadium dioxide (VO2 ), which based on PET/vanadium dioxide fabricated by transfer printing technology (VO2 )/PDMS multilayer structure. De VO2 layer deposited by polymer-assisted deposition (PAD) technology is etched and attached to the PDMS film. After stretching, nano-type spider web cracks formed, and then the layer press to the flexible PET substrate. It can detect the temperature in the range of 270–320 K. The temperature sensing performance with a resolution of 0.1 K attributed to the high TCR of the VO2 materiaal. The collected temperature signal and the mechanical signal are separated through the algorithm's difference to achieve simultaneous monitoring the effect of temperature and mechanical changes. In order to solve the problem of the flexible temperature sensor's insufficient stretchability, Yu et al. [99], based on transfer printing technology, invented a flexible device that can maintain the sensor performance even when the flexible device is stretched or compressed by 30%. They used the Au/Cr layer as a thermistor to patterned on SOI through standard photolithography technology. The peeled heat-sensitive layer adhered through a flexible PDMS stamp, and then transferred and printed on a pre-stretched flexible PDMS substrate to release the substrate. The fabricating process of forming a stretchable flexible temperature sensor, as shown in the Fig. 7a.
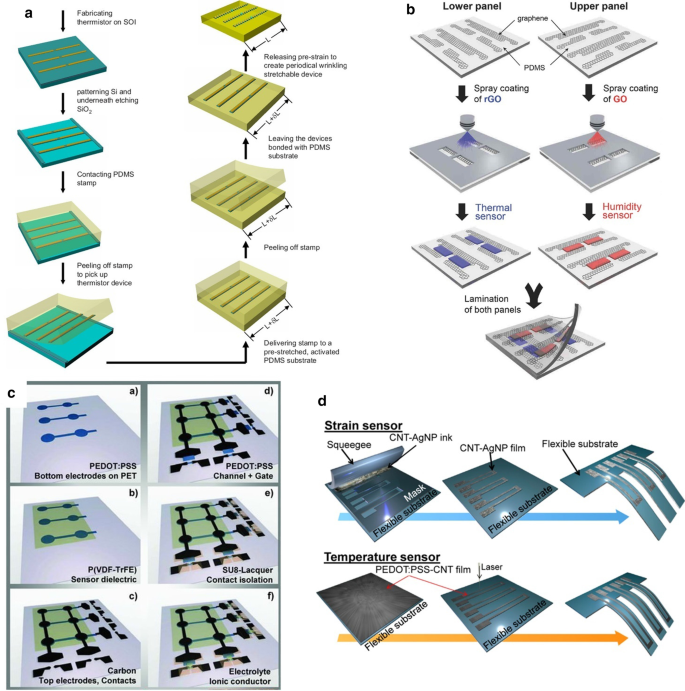
Fabrication method of flexible temperature sensor. een Schematic illustration of the fabrication process [99]. b Fabrication of the stretchable and multimodal all-graphene E-skin sensor matrix [84]. c Process flow illustrating the fabrication of printed ferroelectric active matrix sensor arrays [169]. d Multifunctional e-whisker fabrication [11]
Inkjet printing is an accurate, fast, and reproducible thin-film fabricating technology, which has been widely used in sensor development. Compared with other printing methods, inkjet printing has the advantages of convenience, flexibility, rapidity, low cost, compatibility, accuracy, etc. [166,167,168]. The patterns of inkjet printing need to be post-processed (drying, curing, sintering, etc.) to be fully formed. Improve the performance of printed patterns by converting ink nanoparticles into continuous materials. The properties of the surface tension and viscosity of the ink during the printing process, the quality of the printed pattern also places high requirements on the performance of inkjet equipment [97]. Under the condition of a specific size of the substrate, the conductive track's length formed as long as possible, and the thickness, width, and spacing of the track are reasonable. Repeated experiments obtain the ejection coefficient of the inkjet system. For example, Dankoco et al. [102] used the ink printing method deposit a composite ink with silver as the main component on the PET film to make a flexible and bendable temperature sensor. The circuit on the substrate is clear and smooth, and the ink drops are consistent, which used to measure human body temperature. The picture shows the fabricating process of the extremely sensitive and transparent multifunctional electronic skin sensor matrix developed by Oh et al. [108] The flexible array has the function of monitoring temperature, humidity, and strain. It can feel sensations, such as breathing and touch. GO and rGO, which used as humidity and temperature sensing materials, are sprayed on the PDMS substrate of the graphene circuit grown by the CVD method through inkjet printing technology through a mask. The two sensors are horizontally and vertically aligned, and the temperature sensor is on the bottom layer (as shown in Fig. 7b). After cross-lamination, a PDMS/graphene pressure strain sensor is formed. As a multifunctional flexible sensor, it can collect at the same time but independently respond to a single signal. Inkjet-printed graphene is seven orders of magnitude higher than CVD-grown graphene. The performance advantages reflected in many articles, and some research results are better than CVD-made graphite products. Inkjet printing and screen printing are both rapid and low-cost technical means to realize large-area sensor fabricating. Zirkl et al. [169] combined the two rapid fabricating technologies to create a fully printed flexible sensor array that uses multiple screens. In printing and inkjet printing, only five functional inks used to easily integrate multiple functional electronic components (including pressure and temperature-sensitive sensors, electrochromic displays, and organic transistors) on the same flexible substrate (in Fig. 7c). Because the fabricating speed and low cost of the process can also be applied to the smart sensor network using the roll-to-roll (R2R) fabricating process in the future. The development of a disposable electronic skin system (EES) is particularly critical. Similar to the previous example. Vuorinen et al. [56] introduced a temperature sensor similar to a band-aid after inkjet printing. The sensor uses graphene/PEDOT:PSS composite ink and the printing done on PU material suitable for skin. In particular, in addition to being able to achieve a sensitivity higher than 0.06% \(^{ \circ } {\text{C}}^{ - 1}\), they used inkjet printers to perform serpentine patterned inkjet printing between the silver screen printed with a high resolution (1270 dpi) improved the lack of inkjet printing for printing complex graphics such as snakes. With the use and research of inkjet technology in flexible electronics, the technology for controlling nozzles and inks is also improving. Compared with the speedy fabricating process of screen printing, inkjet printing needs to go through a debugging process, and the printing speed is not as good as screen printing. Also, the small number of nozzles running simultaneously and the high nozzle failure rate limit the inkjet printing fabricating technology to laboratories, and the large-area fabricating requirements of industrial production cannot achieve.
Laser direct writing (LDW) technology uses calculations to design pre-designed patterns. It directly uses laser beam ablation without masking and vacuums deposition. It can directly complete pattern transfer on the surface of the substrate material, with good spatial selectivity and high direct writing speed and processing accuracy, short cycle, high material utilization, and low pollution. Compared with traditional temperature thermistors that require high temperature and a variety of complex processes to activate the sensing function, LDW can achieve selective annealing on a predetermined pattern. A novel integral laser induced by Shin et al. [170] The laser-induced reduction sintering (m-LRS) fabricating scheme can also reduce metal oxide NPs during the annealing process. Scratch NiO NPs ink on a fragile PET substrate, and use m-LRS technology to directly reduce NiO to pattern a linear Ni electrode to form a planar Ni–NiO–Ni heterostructure (as shown in Fig. 8a). The unique method of fabricating a complete flexible temperature sensor system composed of Ni electrodes and NiO sensing channels from a single material NiO NPs provides a new idea for the rapid fabricating of flexible temperature sensors. Different from the previously designed and fabricated fully-printed sensor arrays, Harada et al. [11] proposed a more direct method of mass-fabricating flexible temperature sensors based on previous research. The flexible temperature sensing composite ink printed on the substrate, using the laser for etching away the excess part directly, leaves the designed pattern on the substrate and completes the fabricate of the temperature sensor array. Cut the base to imitate the animal's whiskers to create an artificial electronic whisker (e-whisker) structure (Fig. 7d), strain sensor formed by laminated screen printing. Bionic sensor arrays can scan and perceive three-dimensional objects by using structural advantages.
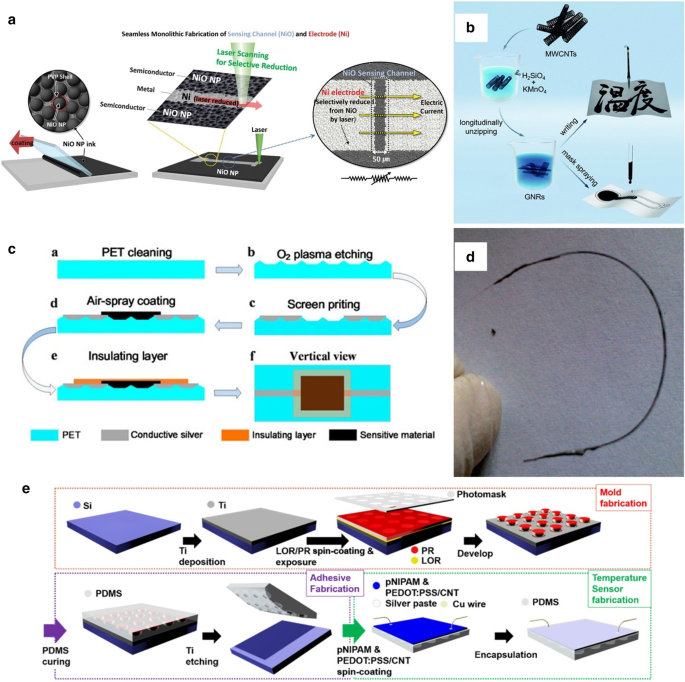
Commonly used fabricating methods. een Schematic of the m-LRS process [170]. b Schematic diagrams showing fabrication process of the paper-based GNRs sensors [171]. c Fabrication process of the temperature sensors [87]. d Flexible temperature sensor on yarn, contacts made of polymer conductive paste [111]. e Fabrication process for the mold, adhesive, and temperature sensor [108]
Under the premise of realizing low-cost and large-area fabricating, disposable intelligent monitoring equipment not only allows monitoring behavior to use anytime and anywhere but also ensures the safety and hygiene of the flexible monitoring equipment. Gong et al. [171] proposed a pen-on-paper fabricating method that uses a brush write or a mask to graphene nanoribbon (GNR) conductive ink dripped between the carbon nanotube electrodes on the paper base. Shown in Fig. 8b. The flexible temperature sensor fabricated after encapsulation with transparent tape. In their research, GNRs have excellent sensing performance and meet the requirements for body temperature monitoring. The fabricating method provides reliable support for the application of fast, large-area disposable flexible equipment.
Other Commonly Used Fabricating Methods
Spin-coating the base layer, active layer, and encapsulation layer by layer is a common way to fabricate flexible temperature sensors by the liquid phase method. Spin coating technology often used for substrate fabricating and packaging protection. For solution-like sensitive materials, the spin coating used. The coating can not only significantly improve production efficiency but also has excellent potential for large-area fabricating. Kim et al. [172] demonstrated the fabricating process of an organic field-effect transistor (OFET) array, using a spin-coating process to design a microstructure on a mold with a crystallized P(VDF-TrFE) material as a gate dielectric and other materials. Material packaging forms OFETs, and the introduction of microstructures enhances the sensing performance. The fabricating process of the bionic structure's [108] flexible temperature sensor fabricated. The adhesive layer of the bionic octopus foot sucker structure is fabricated by a spin coating process, combined with photolithography peeling technology and inverted mold technology (in Fig. 8e). It has an excellent temperature monitoring effect, and the materials used also perform well in terms of human biocompatibility. Liu et al. [87] After surface ionization treatment on the substrate, the flexible electrode was screen-printed, and then the sensitive layer rGO was connected to the flexible wire through the manufacturing method of air-spray coating, and the main structure of the temperature-sensitive flexible sensor was formed after packaging (see in Fig. 8c). It is possible to make robot skins. Huang et al. [68] fabricated a temperature sensor with flexibility, high resolution, and high repeatability in the temperature range of 25–42 °C. They dropped the PEO1500/PVDF/Gr composite solution after stirring and ultrasonic treatment on a polyimide flexible substrate. It was spin-coated at a certain speed to form a layer. It packaged it into a temperature sensor, which verified the medical body temperature transmission sense of possibility and feasibility. Spin-coating technology as an effective fabricating process for wearable devices has also introduced into Wu et al. research [173]. They designed an organic thin-film transistor with heat-sensing ability. Cleverly through the unique three-arm three-dimensional composite polylactide, polylactic acid (tascPLA) solution is spin-coated on the Au gate electrode evaporated on the Si substrate. Then the Au source and drain are deposited on it by a thermal evaporation method. The composite film containing tascPLA also serves as the gate of the OFET dielectric and substrate materials. In flexible electronics, there are not a few that use fabric as the carrier of the sensing element. The dip-coating of conductive yarn/fabric in conductive ink is the most commonly used coating technology for flexible sensors. The development of conductive fabrics provides a reasonable prerequisite for the application of smart fabrics. Sibinski et al. [111] developed a temperature sensor that monitors the temperature range of 30–45 °C. Moreover, it currently realizes filamentary miniaturization on a single yarn. In Fig. 8d shown, the PVDF monofilament fiber is coated with PMMA polymer compound mixed with multi-walled carbon nanotubes (MWCNTs) as a heat-sensitive layer by dip coating technology has good temperature sensitivity and extremely high repeatability. It is used as a fabric easily integrated into knit clothing. Another method, based on the molding properties of organic materials, is also used to fabricate flexible sensor membrane structures or to fabricate samples for testing sensing performance. The fabricating process of the thermoelectric nanogenerator (TENG) studied by Yang et al. [107] The drop-casting film method, after comparison, discards the PEDOT:PSS material, which has a weak drop-casting effect and is easy to break, not only the mixture of Te-nanowires and P3HT polymer in chlorobenzene solution is drop-casted on the flexible Kapton substrate to make a composite film, the composite material is also cast on the white fabric cloth, which also has apparent temperature sensing ability and thermoelectric performance, which can be used in wearable heat collectors. Rich and diverse fabricating methods lay the foundation for the development of flexible electronics. Under the requirements of large-scale and large-scale production, suitable fabricating technology is also continually developing. In the future, perfect wearable flexible devices are expected.
Resultaten en discussie
Key Parameters of Flexible Temperature Sensor
The flexible temperature sensor can be attached to the human body to monitor the subtle temperature changes on the surface of the human body or real-time temperature monitoring of specific parts, and even the temperature of the core or tissues and organs in the body. They respond to temperature changes by changing the resistance and output the temperature changes as electrical signals. With the increasing demand for flexible electronic devices, the disadvantages of traditional temperature sensors, such as poor scalability, inability to carry, and poor real-time performance, are becoming increasingly unsuitable for flexible wearable devices. Today flexible temperature sensors are required to have high performance, such as high sensitivity, fast response time, reasonable test range, high precision, and high durability to realize the monitoring function better.
Sensitivity
Sensor sensitivity refers to the ratio of the change ∆y of the system response under static conditions to the corresponding input change ∆x , that is, the ratio of the dimensions of output and input. When the sensor output and input dimensions are the same, the sensitivity can be understood as the magnification [21, 174, 175]. The temperature coefficient of resistance TCR (TCR, in \(^{ \circ } {\text{C}}^{ - 1}\)) of the common resistance type flexible temperature sensor is expressed in the following expression, \(\Delta R/R_{0} =s\left( {T - T_{0} } \right)\), is the relative resistance change (\(\Delta R/R_{0}\)) as a function of temperature, where \(s\) represents TCR. If the sensor output and input show a linear relationship, the sensitivity is constant [83]. Otherwise, it will change with the input quantity. Generally speaking, by increasing the sensitivity, higher measurement accuracy can be obtained. Most flexible sensors used for body temperature monitoring only pay attention to temperature changes within 10 °C and therefore require high-temperature sensitivity to capture relatively small temperature changes. Here, several typical methods and concepts for improving the sensitivity of flexible temperature sensors are summarized to provide a favorable reference for further improvements.
The sensing performance of flexible temperature sensors is usually closely related to the properties of materials. One possible way to realize the development of sensitive sensors is to adopt composite materials with visible thermal performance. After temperature stimulation, the internal conductive network will change, which will lead to affect the temperature sensitivity of the device. To improve the temperature sensitivity of the active material, a strategy that converts temperature fluctuations into mechanical deformation is adapted to amplify the response of the conductive network to temperature changes. The thermosensitive material is connected to a substrate with a high positive thermal expansion coefficient to enhance thermal induction deformed. This method has applications in sensors made of graphene, graphite and graphene-nanowalls. For example, in the flexible temperature sensor designed and fabricated by Huang et al. [68], Gr establishes a conductive path in the PEO/PVDF binary composite material. In the process shown in the Fig. 9b. The temperature change will cause the PEO to transform from crystal to amorphous, and melt when the temperature is close to the melting point of PEO, the volume expansion of PEO will destroy the conductive network in the composite material, resulting in a sharp increase in resistivity-increasing the strength of the PTC, which can quickly reaction within a narrow temperature range change. Experiments show that PEO thermal performance plays a leading role in the PTC effect of the device. Another way to improve the sensitivity of a flexible temperature sensor is to use a unique structure. In recent years, to improve temperature sensing performance, various design strategies have been developed by changing the structure. Yu et al. [176] recently proposed a method based on engineering microcrack morphology to change the crack morphology of the PEDOT:PSS film on the PDMS substrate by adjusting the substrate surface roughness, acid treatment time, and pre-stretching degree to improve the temperature sensitivity of the sensor. Figure 9d shows the effect of average crack length and cracks density on temperature sensitivity. The result is that the longer the crack length, the higher the crack density, and the higher the temperature sensitivity. It is proved that the micro-crack structure plays a vital role in the temperature sensitivity of the sensor. It is verified that obtaining a high-density micro-crack structure is the key to obtaining the high-temperature sensitivity of the sensor. High density and high length directly correspond to higher temperature sensitivity. The fabricating process of flexible electronic equipment plays a vital role in the production of the device and can effectively improve the sensitivity of the flexible temperature sensor. Just as Shin et al. [170] used a rapid overall laser-induced reduction sintering (m-LRS) method for fabricating Ni/NiO flexible temperature sensor is different from the inkjet printing method. They directly reduce and sinter the Ni electrode on the NiO layer, and form a high-quality overall contact between the metal electrode (Ni) and the temperature-sensitive material (NiO). Ni–NiO–Ni heterogeneous temperature sensor shows higher temperature sensitivity than other sensors of the same type (Fig. 9e). Raman spectroscopy and X-ray diffraction (XRD) measurements show that the superior sensitivity comes from the unique thermal activation mechanism of the m-LRS process. Besides, since flexible temperature sensors are mostly touch-sensitive, it is inferred that the film's thickness affects the sensitivity. The study of Lee et al. [177] verified the effect of the thickness of the highly sensitive paper base on the sensitivity, they use a simple dipping fabricating method to deposit sensitive materials on the printing paper. Dipping time will affect the thickness of the film. The Fig. 9f also shows the different performance of sensitivity due to different thicknesses. It should be noted that the higher the sensitivity, the narrower the measurement range and, worse, the stability. Therefore, it is necessary to pay attention to increasing stability and accuracy while improving sensitivity.
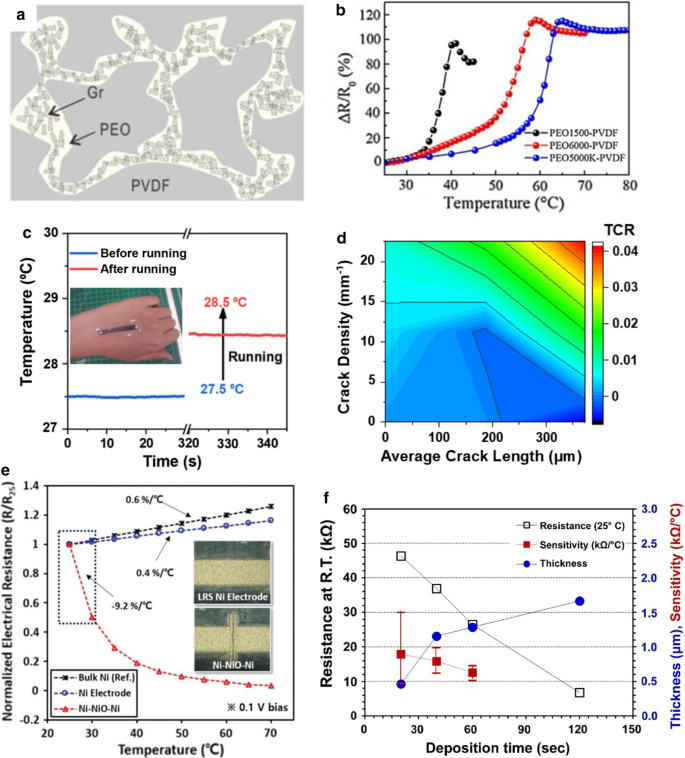
Sensitivity of flexible temperature sensor. een Images of the PEO1500/PVDF/Gr at room temperature. b Resistance curves of PEO1500/PVDF/Gr, PEO6000/PVDF/Gr and PEO5000K/PVDF/Gr composites versus temperature [68]. c The temperature measurement results before and after exercise, the illustration shows the flexible temperature sensor attached to the back of the hand. d Heatmap as functions of average crack length and crack density for TCR valuses from all developed sensors [176]. e PTC and NTC characteristics of the m-LRS processed Ni electrode and Ni–NiO–Ni structure. Inset digital images are m-LRS processed Ni electrode (upper) and Ni–NiO–Ni structure (lower) [170]. v The results of the deposited film thickness (blue circle), resistance at room temperature (white square) and sensitivity (red square) as a function of deposition time [177]
Other Parameters of Flexible Temperature Sensor
The sensing range of a flexible temperature sensor is an important parameter, which refers to the minimum and maximum temperature that can be detected. In this article, we are only interested in the measurement range (30–45 °C) suitable for body temperature monitoring. Another vital performance parameter, response time, is generally defined as the time consumed by the temperature sensor from applying temperature stimulation to generating a stable signal output. Some documents also define the temperature sensor's response time by the time the sensor temperature rises from \(T_{{{\text{sensor}}}}\) to 90% of the temperature rise (\(T_{0}\)) [145]. It is related to the thermal response of the active material itself and reflects the rapid response ability of the temperature sensor to temperature. In terms of applications, such as real-time human body health-monitoring products and wearable artificial intelligent elements with an instant response, all have a shorter response time.
The sensor ability to sense the smallest amount of change to be measured is defined as resolution. In other words, the input quantity starts to change from a non-zero value. At this time, if the input change value does not exceed an absolute value, and the sensor's output does not change, it means that the sensor cannot distinguish the change of the input quantity. Accuracy refers to the ratio of the value of plus or minus three standard deviations near the real value to the range, the maximum difference between the measured value and the real value, and the degree of dispersion of the measured value. For measuring instruments, accuracy is a qualitative concept and generally does not require numerical expression. Because the normal fluctuations of human body temperature within a day are small, high resolution or high accuracy is significant for flexible temperature sensors for body temperature monitoring, determining the broader application of flexible temperature sensors.
Repeatability is the degree of inconsistency of the measurement results for the same excitation quantity when the measurement system performs multiple (more than 3) measurements on the full range in the same direction under the same working conditions. In a flexible temperature sensor, durability means that it maintains a stable sensing function and a complete device capability under a long-term use environment. A flexible temperature sensor with high durability and high repeatability can meet the basic requirements of long-term stable use. Linearity usually defines as the degree of deviation between the actual input–output relationship curve and the ideal fitting curve, usually expressed as a percentage. Therefore, in the linear range, the output signal will be more accurate and reliable. High linearity is also conducive to the input–output signal calibration process and subsequent data optimization processing [58]. The demand for the development of flexible temperature sensors with high linearity is also increasing.
Applications
The ability to live organisms to respond accurately and quickly to external environmental stimuli is an essential feature of life. The induction of temperature allows humans to predict dangers and respond to diseases. Abnormal changes in body temperature often play a crucial reminder and help for early prevention [179]. With the research and development of flexible electronic devices, such as electronic skins, smart health monitoring systems, smart textiles, and biomedical equipment, sensors with the multi-functional signal acquisition are necessary [180, 181]. Among them, the flexible temperature sensor is an indispensable and vital part of medical and health applications [96]. In the past few years, the progress of new materials, new fabricating technologies, and unique sensing methods have provided an essential reference and a solid foundation for the development of a new type of skin-like flexible temperature sensor. In these sensors, some basic performance parameters such as sensitivity, resolution, and response time even exceed natural skin. Although, after a lot of basic and applied research, research results can be transformed from the laboratory to real-world use. However, there are still many challenges waiting to be solved in practical applications. In the following content, we will summarize recent flexible temperature sensors' results in flexible electronics applications with unique, excellent, and practical application examples.
Flexible sensors have the characteristics of large-area deformability, lightweight, and portability, which can realize functions that traditional sensors cannot. Electronic skin integrates various sensors and conductors on a flexible substrate to form a highly flexible and elastic sensor similar to the skin. It converts external stimuli such as pressure, temperature, humidity, and hardness into electrical signals and transmits them to the computer for processing, even can recognize regular objects [14]. An electronic skin with similar functions is a necessary feature of future robots to achieve perception in an unstructured environment. The electronic skin enables the robot to perceive changes in the external environment as sharply as a real person. Although the principle of electronic skin is simple, there are still challenges in covering the surface of the robot with electronic skin. The use of the electronic skin determines, that is, maintaining the device's integrity while maintaining the sensing performance during mechanical deformation. The ideal method of flexible electronic skin is patterned fabricating. Using solution materials, directly deposited on the substrate through printing technology to form patterns, and achieve roll-to-roll (R2R) large-area fabricating under normal temperature and pressure so that the skin has the advantages of considerable size, high yield, low production cost, and environmental protection. Someya et al. [182] have developed an electronic mesh skin with flexibility, large area, integrated temperature, and pressure-sensing capabilities, as shown in the figure. The stretchable electronic skin has multiple heats, and pressure sensors distributed at the nodes and read data through OTFT. Among them, the thermal sensor array is based on organic diodes, prepared on polyethylene naphthalate (PEN) coated with indium tin oxide (ITO) on the surface. The thermal film is mechanically cut by a numerical control cutting machine, and the R2R process prepares the network structure, and then the network film is combined by lamination to complete the preparation of the thermal sensor array. The parylene protective layer is placed on the organic semiconductor layer to act as a flexible gas barrier layer, extending the device's durability from days to weeks and avoiding mechanical damage to transistors and diodes during testing. When the sensor is in its original state, the space is square, and it becomes a rhombus after being stretched, and it can still maintain excellent electrical characteristics when stretched 25% (shown in Fig. 10a). The establishment of the mesh structure expands the use of electronic skin. The distributed structure of multiple parameters and multiple nodes also makes it possible to monitor irregularly shaped objects. A kind of epidermal electronics proposed by Kim et al. [53] refers to ultra-thin flexible electronic devices fixed on the skin's surface (in Fig. 10b). Only through van der waals force can it fit perfectly with the skin and sense the temperature, strain, and dynamic response. Potential applications include physiological state detection (electroencephalogram, electrocardiogram, electromyography), wound detection or treatment, biological/chemical perception, human–computer interaction interface, wireless communication, etcetera. Integrating all devices in the measurement device in a completely different way integrates a variety of functional sensors (such as temperature, strain, electrophysiology), micro-scale light emitting diodes (LEDs), active/passive circuit units (transistors, diodes, resistors), wireless power supply coils, wireless radio frequency communication devices (high-frequency inductors, capacitors, oscillator, and antenna). Skin electronics has the characteristics of ultra-thin, low elastic modulus, lightweight, and ductility. The device is fabricated in the form of winding-shaped filament nanowires or micro-nano thin films, enabling the system to withstand more significant elastic deformation. It can be easily transferred to the skin surface through the soft-touch process, just like a tattoo sticker. Although the electronic skin has wealthy functions, the resonance frequency will drift when the strain exceeds 12%. Besides, the durability of ultra-thin flexible devices also requires attention. The shortcomings mentioned above need to be considered when designing and fabricating future electronic skin systems.
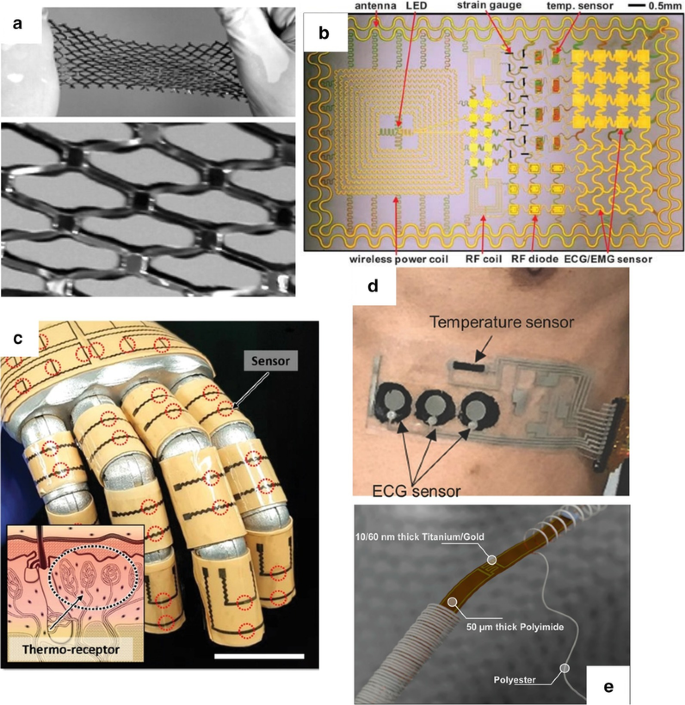
Application demonstration of flexible temperature sensor. een A plastic film with organic transistors and pressure-sensitive rubber is processed mechanically to form a unique net-shaped structure, which makes a film device extendable by 25%. A magnified view of extended net-structures is also shown [187]. b Image of a demonstration platform for multifunctional electronics with physical properties matched to the epidermis [155]. c Model hand covered with the temperature-sensitive artificial skin with enlarged illustration of skin thermos-receptors [170]. d Photo of the fabricated device [184]. e Concept of the flexible temperature sensor embedded within the fibres of a textile yarn [185]
The development of a wearable health monitoring system is for collecting human body thermal parameters:body temperature, epidermal temperature, heat flow [183], etcetera. For observation and inference such as metabolism, fever, disease infection, skin healing, thermal adaptation (implants, prostheses), etc. Compared with the limitations of traditional monitoring systems in the use cases. Flexible wearable temperature monitoring systems have demonstrated more flexible applications and excellent thermal parameter monitoring effects in recent studies—the m-LRS NiO temperature sensor system fabricated by the Shin et al. team that we introduced earlier. In an experiment to verify the potential of its system as an electronic skin application. As shown in the Fig. 10c, three side-by-side temperature sensors attached to the robot finger can make an accurate flow rate of hot or cold water flowing in the microfluidic pipe, flow direction, and temperature response. Besides, it has a high-resolution capability for the temperature increase caused by infrared heat radiation. Moreover, the high-curvature fit feature allows the temperature sensor system also to monitor breathing to provide early warning of abnormal respiratory symptoms caused by disease or poisoning. Experiments on breathing changes before and after exercise have verified the system's effectiveness in monitoring small changes in human breathing and temperature. The holistic fabricating technology they put forward provides useful help for the large-scale development of the healthcare system and electronic skin. Webb et al. [145] reported two types of flexible, ultra-thin, and sensitive temperature sensors/actuators with different structures, namely, a TCR sensor array with a serpentine network formed by Cr/Au fabricated by microlithography technology and a TCR sensor array after deposition. Corrosion nano PIN sensor, the two sensors have high sensitivity, rapid response, and high precision. Non-invasive monitoring of the subtle changes in skin surface temperature caused by changes in human blood flow caused by external stimuli can be realized. By accurately measuring skin thermal conductivity and monitoring skin moisture, it has practical application value for health management such as blood sugar monitoring, drug delivery and absorption, and wound or malignant tumor changes. For a flexible sensor system that needs to monitor health status based on vital signals, easy fabricating, secure attachment, and strong biological adaptability are essential. As Fig. 10d shown, Yamamoto et al. [184] developed a gel-free viscous electrode that is more suitable for electrocardiogram (ECG) sensors. A temperature sensor fabricated by patterned printing is assembled to form a flexible sensor system that can monitor the occurrence of dehydration or heatstroke symptoms. The use of biocompatible materials solves the problem that traditional gels cannot be attached to the human body for a long time. The open, flexible sensing system platform can also add various sensors to develop a broader range of applications. The temperature and heat transfer properties of the skin can be characterized as critical information for clinical medicine and basic research on skin physiology. In terms of thermal adaptation, it is crucial to monitor and predict the residual limb's skin temperature. For example, the prosthesis gaps create a warm and humid micro-environment, which promotes the growth of bacteria and causes inflammation of the skin. Local skin temperature monitoring uses flexible temperature sensors hidden in daily textiles. Due to its high consistency, it is very suitable for non-existent monitoring of skin temperature, which significantly benefits patients and medical staff. Lugoda et al. [185] used different industrial yarn fabricating processes to integrate flexible temperature sensors into fabrics, and studied the sensing effect of embedding flexible temperature sensors in textile yarns. The bending resistance and repeatability of the temperature sensor embedded in the yarn are verified. The elongated sensor is made by patterning a Ti/Au layer deposited on a PI film (see in Fig. 10e). Experiments with the temperature sensor embedded in the armband have verified that this sensor yarn can be used to fabricate intelligent temperature-measuring textile garments. Smart temperature measuring textiles can be used for thermal adaptation monitoring applications such as the detection of thermal discomfort in prostheses, socks for early prediction of diabetic foot ulcers [186], textiles that continuously measure the body temperature of babies, and bandages for monitoring wound infections. The use of industrial yarn fabricating technology to integrate flexible temperature sensors makes large-scale smart textile fabricating possible.
With the development of flexible electronics, the application of multifunctional flexible wearable devices in health care, smart monitoring equipment, human–computer interaction/combination, smart robots, and other related fields have become more and more extensive. Flexible temperature sensors have strong practicability in flexible wearable electronic devices, especially for monitoring human health, which is inseparable from accurate temperature control. The introduction of the wireless transmission function allows people to analyze and utilize the temperature sensor's measurement results. Honda et al. [133] proposed a wearable human–computer interaction device, a patterned "smart bandage" with drug delivery function. The device uses low-cost patterned printing technology to integrate temperature sensors, MEMS-structured capacitive tactile sensors, and wireless coils on a flexible substrate. Figure 11a shows the physical object of the flexible device on the arm. A drug delivery pump (DDP) made by soft lithography and a microchannel for drug discharge can be added to supply drugs to wounds or internal parts to improve the wearer's health. As a wearable interactive humanized health monitoring wireless device application, it will play a positive role in future wearable electronic applications. Intelligent wound patch:wounds after trauma are infected and difficult to heal. Under traditional wound dressings, the healing process of wounds is often unpredictable, and satisfactory repair results cannot be obtained. In wound infection or healing, temperature changes different from the normal body temperature will occur. Therefore, monitoring the subtle temperature changes at the wound is quite necessary for ideal wound healing [188]. To eliminate the "black box" state in the wound healing process and grasp the wound's situation in real-time, Lou et al. [189] proposed a transparent and soft, closed-loop wound healing system to promote wound healing real-time monitoring of the wound. The flexible wound healing system (FWHS) and flexible wound temperature sensing device (FWTSD) likes the form of band-aids, their flexible device in the shape of a double-layered band-aid can be directly attached to the wound. The upper layer is a flexible temperature sensing layer composed of temperature sensors, power management circuits, and data processing circuits. The lower layer is a collagen-chitosan dermis equivalent for skin regeneration. A customized software application (app) installed on the smartphone receives data from the sensing layer, displaying and analyzing wound temperature in real-time (Fig. 11b). The system has high sensitivity and stability, good ductility, reliability, and biocompatibility. In addition to monitoring the normal wound regeneration process, in the biological experiments, timely warning of severe wound infection was achieved. A smart wound healing system with growth promotion, real-time temperature monitoring, wireless transmission, and visualization that integrates wound monitoring, early warning, and on-demand intervention may occur in the future. If the wound after an injury is severely infected and is not treated in time, more serious damage to the body may occur. Timely monitoring of wound conditions and rapid, effective diagnosis and treatment are urgent needs to reduce the occurrence and aggravation of wound infection complications. In another study, Pang et al. [190] proposed a flexible smart bandage with a double-layer structure with high sensitivity, good durability, and remote control. The upper layer is packaged in PDMS connected and integrated through a serpentine wire. The temperature sensor, ultraviolet (UV) light-emitting diode, power supply, and signal processing circuit are designed, and the lower layer is designed with a UV-responsive antibacterial hydrogel. When the temperature sensor detects an abnormal temperature exceeding the threshold, it will wirelessly transmit the signal to the smart device. Moreover, control the in-situ ultraviolet radiation through the terminal application to release antibiotics from the underlying hydrogel and apply it to the wound to achieve early infection diagnosis and processing. The system can monitor wound status in real-time, diagnose accurately, and treat on-demand. The research of this intelligent trauma diagnosis system provides new strategies and diagnostic and treatment ideas for the prevention and treatment of traumatic diseases such as wound treatment and diabetic ulcers. The popularization and application of such systems also have significant prospects. The development of advanced surgical tools is an important measure to improve human health. The advancement of flexible electronics provides reliable support for the flexibility and miniaturization of clinical medical instruments. Simultaneously, the function of flexible devices is enriched, allowing clinical instruments to perform multiple operations on diseased parts in a short period, reducing the risk of surgery. In Fig. 11c,d, Kim et al. [191] invented a direct integration of a multifunctional element group, including a flexible temperature sensor array with the elastic film of a traditional balloon catheter, which provides a variety of functions for clinical applications. The use of this balloon catheter in live animal experiments verified the instrument's ability to provide key information about the depth of the lesion, contact pressure, blood flow or local temperature, and the radiofrequency electrodes on the balloon for tissue controlled local ablation. Specific use in cardiac ablation therapy. In another study, they developed two thin and bendable flexible temperature sensor applications. Used for medical sutures, one of the simplest and most widely used devices in clinical medicine. One is an ultra-thin suture line based on the integrated Au thermal actuator and silicon nanodiode temperature sensor on the biofiber membrane. The other suture line is two \(1 \times 4\) Pt metal temperature sensor arrays formed between Au wires on both sides of the substrate. To achieve the purpose of helping biological wounds heal and monitoring wound recovery. It is full of challenges to build semiconductor devices and sensors and other components on a biologically adaptive platform that touches the flexible curved surface of the human body.
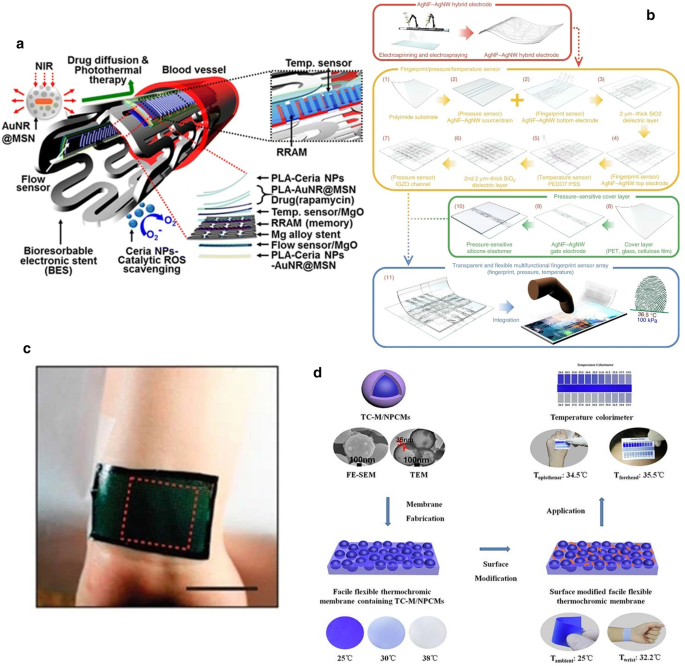
Application demonstration of flexible temperature sensor. een Photos of the smart bandage integrated with touch and temperature sensors, a wireless coil, and a DDP [133]. b The application scenario of FWHS. The FWTSD with a Band-Aid shape tightly contacts with the wound site. Temperature variation is detected by temperature sensor [189]. c Optical image of a multifunctional balloon catheter in deflated and inflated states [191]. d Optimized mechanical structure shown in a schematic illustration when wrapping [53]. e Image of bioresorbable pressure and temperature sensors integrated with dissolvable metal interconnects and wires. v Diagram of a bioresorbable sensor system in the in a rat's skull [193]
As we all know, some operations will leave objects different from the body's tissues such as steel plates, stents, pacemakers, implants, etcetera, inside the body, which will cause discomfort to the body [192]. The development of absorbable devices will optimize surgical implantation equipment Post-access problems reduce the risk of surgery and the difficulty of post-wound healing. Kang et al. [193] reported a multifunctional silicon sensor. The experiment of implanting the sensor in rats' brains proved that all the materials (polylactic-glycolic acid, silicon nanomembranes (Si-NMs), nanoporous silicon, SiO2 ) constituting the sensor could be used. Naturally absorbed through hydrolysis and/or metabolism, no need to extract again. It can continuously monitor parameters such as intracranial pressure and temperature, which illustrates the performance advantages of absorbable devices for treating brain trauma. The emergence of implantable vascular stents provides strong support for the smooth progress of interventional surgery. The vascular stent can expand the blood vessel through a continuous structure in the blocked blood vessel to restore blood flow. However, the inflammation caused by traditional vascular stents in the body for a long time is still difficult to be diagnosed and cured. Son et al. [194] introduced the bioabsorbable electronic stent (BES) applications based on bioabsorbable and bioinert nanomaterials.(Fig. 11e,f) Integrated flow sensing, temperature monitoring, wireless power/data transmission, and inflammation suppression, local drug delivery, and hyperthermia device. The Mg temperature and flow sensor are composed of an adhesion layer, a fiber-shaped Mg resistance (sensing unit), and an outer encapsulation layer (MgO and polylactic acid(PLA)) (as shown in Fig. 12a). Experimental studies have shown that combining bio-absorbable electronic implants and bio-inert therapeutic nanoparticles in intravascular smart stent systems has not yet been recognized.
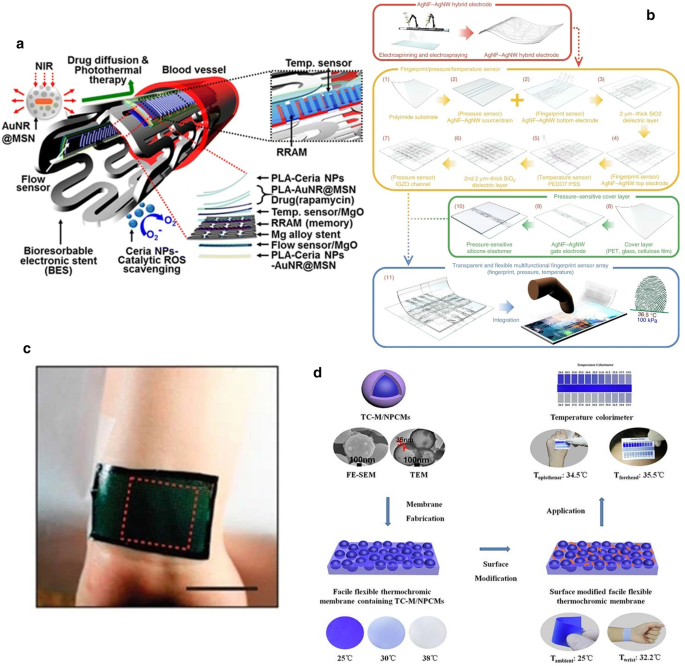
Application demonstration of flexible temperature sensor. een Schematic illustration of the BES (left), its top view (top right), and the layer information (bottom right). The BES includes bioresorbable temperature/flow sensors, memory modules and bioresorbable/bioinert therapeutic nanoparticles [194]. b The fabrication process for the multiplexed fingerprint sensor [196]. c Optical images of an e-TLC device on the wrist during an occlusion test (scale bar, 3 cm) [15]. d Facile flexible reversible thermochromic membranes based on micro/nanoencapsulated phase change materials for wearable temperature sensor [198]
Temperature visualization makes the function of flexible temperature sensors more prominent. It makes up for the disadvantages of traditional infrared imaging equipment that are expensive, unfavorable for carrying, and inaccurate in measuring dynamic objects. The appearance of high-intensity focused ultrasound (HIFU [55]), smart device monitoring, and other occasions will make temperature measurement more accurate and useful [195]. An et al. [196] developed a PEDOT:PSS temperature sensor with ultra-long silver nanofibers (AgNFs) and silver nanowires (AgNWs) hybrid network as a high-performance transparent electrode, layer, and patterned fabricating, using multiplexing technology to create a capacitive flexible and transparent multifunctional sensor array (Fig. 12b). The capacitance change is 17 times higher than that of a pattern sensor that also uses ITO electrodes. In the future, the visual display of fingerprints, pressure, and temperature changes between the fingers on the flexible sensor can be realized simultaneously on smart devices. Applying in the fingerprint recognition function, the security function of smart mobile devices can improve more security. Gao et al. [15] introduced a light-emitting device that combines a colorimetric temperature indicator with wireless, patterned, and stretchable flexible electronic technology. A large-scale thermochromic liquid crystal (TLC) pixel array is formed on a thin elastic substrate in combination with colorimetric reading and radio frequency (RF) drive to map the thermal characteristics of the skin. Under the premise of non-invasiveness, the sensor system uses radio frequency signals to control local heating to survey and map skin temperature with high-temperature accuracy (50 mK) and high spatial resolution. The blood flow under the skin is evaluated by the reactive hyperemia test (see in Fig. 12c). Also, hydration analysis reflects skin health problems. The figure verifies that the device can quickly visually respond to small changes in blood flow. Reflect cardiovascular and skin health problems through hydration analysis. Like the fluoroscopy capability of an infrared camera, the device can be used for core temperature and wound healing monitoring, near-body implant device inspection, malignant tumor cancer screening, and other biomedical-related functions. The unique feature is that the flexible device can be read using a mobile phone, and it can be worn for a long time. The temperature visualization system provides a massive prospect for the description of the thermal properties of the skin. It makes efforts to provide useful indicators for determining the human health and physiological state. In another study, Kim et al. [197] provided a feasible idea using thermochromic materials to observe temperature changes directly. The difference is that their description of the temperature change does not have a specific numerical display, and can only judge the approximate temperature change range. However, as shown in Fig. 12d, He et al. optimized the accuracy of the mapping temperature of thermochromic materials, which can reflect the specific value of the temperature more accurately [198]. In future research, the development of thermochromic materials sensitive to temperature and can accurately respond to temperature will also gradually becoming one research focus. In particular, the reverse cushioning material they made shown a color similar to human skin. Besides, they used 3D printing technology to fabricate the flexible pressure sensor, simplifying the fabricating process, because the sensor structure parameters can be adjusted, showing customizable functions, and achieving the purpose of overall structural fabricating. In the future, 3D printing is the best candidate for the development of unique functional structures [199]. Because this technology can achieve the overall fabricating of the device, it simplifies the fabricating process and ensures integrated molding requirements. It will also gain enormous popularity in the field of flexible sensors.
Conclusies
This article reviews the recent research progress of high-sensitivity flexible temperature sensors in human body temperature monitoring, heat-sensitive materials, fabricating strategies, basic performance, and applications. As a relatively stable dynamic variable in the human body, body temperature or local temperature (trauma) may have different degrees of small fluctuations (about 0.5 °C) under the influence of emotions or physiological activities. The monitoring temperature of the flexible temperature sensor needs to be comparable. Traditional infrared cameras have smaller temperature resolution (< 0.1 °C). Besides, timely and fast monitoring of body temperature is another key to breakthrough. The current temperature sensor response time can be within a few milliseconds, but there is a problem of too long reset time. How to shorten the time difference between response time and reset time will directly affect sensor monitoring's efficiency and capability. With the development of multifunctional sensors, how to avoid mutual interference between multiple signals so that the stimuli of the respective responses between the signals can independently respond without mutual interference and accurately output, which has become an urgent problem to be solved and perfected for improving sensor performance. The development of flexible temperature sensors shows us a foreseeable future. In the future, flexible temperature sensors will also achieve large-area low-cost fabricating, high sensitivity, self-supply, visualization, self-healing, biodegradability, and wireless remote sensing transmission [200]. Furthermore, other functions are integrated and put into use. Sorting the collected temperature data into the health big data platform can provide the best help and data support for human future medical diagnosis. Also, patterned micro-nano fabricating technology is a good suggestion for low-cost mass production of sensors. Based on the relatively mature process flow of the printing process, the realization of integrated multifunctional large-area flexible devices is just around the corner. The current flexible temperature sensor can achieve higher sensitivity, but some sensors do not eliminate environmental factors' interference on the temperature sensor. In the future, the flexible temperature sensor used for body temperature monitoring can make efforts to combat environmental influences. Although the flexible sensor itself can be fragile and light, it needs to be connected to the power supply circuit and the power supply, which dramatically reduces the overall flexibility. In the future, for flexible temperature sensors that monitor body temperature, with the further optimization of signal acquisition methods, real-time visual data wireless transmission can be realized under more efficient self-powered conditions, which will be a vast improvement for intelligent monitoring systems. The monitoring of body surface temperature is greatly affected by the environment, while the core temperature is relatively stable. The flexible temperature sensor used for body temperature monitoring can be attached to the body surface (forehead, arm, armpit, etcetera.) to monitor the body surface temperature and even fluctuate. The core temperature with a small range can also be measured. Non-implantable flexible sensors require more improvements in wearability, biocompatibility, and durability to meet the needs of a broader range of people and become a flexible application device available to everyone. For intrusive flexible temperature sensors, whether in the process of intrusion or during the use of the sensor, minimizing damage to the body is the primary consideration. Therefore, exploring and developing biocompatible or biodegradable sensing materials and sensors is undoubtedly an improvement direction. There will be no rejection or allergic reactions in the body due to foreign bodies. In the future, flexible temperature sensors will appear on many occasions around us. The exploration of the development of flexible temperature sensors with high performance, easy fabricating, low cost, and wide application range will continue.
Beschikbaarheid van gegevens en materialen
Niet van toepassing.
Afkortingen
- PDMS:
-
Polydimethylsiloxaan
- PI:
-
Polyimide
- PU:
-
Polyurethane
- HUISDIER:
-
Polyethyleentereftalaat
- PVA:
-
Polyvinylalcohol
- PVB:
-
Polyvinyl butyral
- CTE:
-
Coefficient of thermal expansion
- CB:
-
Carbon black
- CNT's:
-
Koolstof nanobuisjes
- TCR:
-
Temperature coefficient of resistance
- EG:
-
Expanded graphite
- Gr:
-
Graphite
- PEO:
-
Polyethylene oxide
- PVDF:
-
Polyvinylideenfluoride
- PEDOT:
-
PSS:Poly(3,4-ethylene dioxythiophene)-poly(styrene sulfonate)
- MWCNTs:
-
Multi-walled carbon nanotubes
- SWCNTs:
-
Single-walled carbon nanotubes
- GO:
-
Grafeenoxide
- rGO:
-
Gereduceerd grafeenoxide
- GNWs:
-
Grafeen
- PECVD:
-
Plasma-versterkte chemische dampafzetting
- TS:
-
Transparent and stretchable
- Au:
-
Goud
- Ag:
-
Zilver
- Cu:
-
Koper
- Pt:
-
Platinum
- Ni:
-
Nickel
- Al:
-
Aluminium
- MEMS:
-
Micro-electro-mechanical system
- Cr:
-
Chromium
- OTFT:
-
Organic thin-film transistor
- NP's:
-
Nanodeeltjes
- PE:
-
Polyethyleen
- PTC:
-
Positive temperature coefficient
- VO2 :
-
Vanadium dioxide
- PAD:
-
Polymer-assisted deposition
- NiO:
-
Nickel oxide
- P3HT:
-
Poly(3-hexyl thiophene)
- PPy:
-
Polypyrrole
- pNIPAM:
-
Poly (N-isopropyl acrylamide)
- SEBS:
-
Polystyrene- block-poly(ethylene-ran-butylene)-block-polystyrene
- PDPPFT4:
-
Poly(diketopyrrolopyrrole-[3,2-b]thieno[2′,3′:4,5]thieno[2,3-d]thiophene])
- PII2T:
-
Poly(isoindigo-bithiophene)
- OSC's:
-
Organic semiconductors
- TENG:
-
Thermoelectric nanogenerator
- DN:
-
Double-network
- DMSO:
-
Dimethylsulfoxide
- P (VDF-TrFE):
-
Copolymer polyvinylidene fluoride
- IR:
-
Infrarood
- FET:
-
Veldeffecttransistor
- BT:
-
BaTiO3
- ZnO:
-
Zinkoxide
- MFSOTE:
-
Microstructure-frame-supported organic thermoelectric
- PVD:
-
Physical vapor deposition
- CVD:
-
Chemische dampafzetting
- AlPcCl:
-
Aluminum phthalocyanine chloride
- RF-PECVD:
-
Radio frequency plasma enhanced chemical vapor deposition
- FCCVD:
-
Floating catalyst chemical vapor deposition
- PEI:
-
Polyetherimide
- LS:
-
Laser writing
- R2R:
-
Roll-to-roll
- EES:
-
Electronic skin system
- LDW:
-
Laser direct writing
- GNR:
-
Graphene nanoribbon
- tascPLA:
-
Three-arm three-dimensional composite polylactide, polylactic acid
- XRD:
-
Röntgendiffractie
- m-LRS:
-
Laser-induced reduction sintering
- NTC:
-
Negative temperature coefficient
- ITO:
-
Indiumtinoxide
- LED's:
-
Light emitting diodes
- ECG:
-
Electrocardiogram
- DDP:
-
Drug delivery pump
- FWHS:
-
Flexible wound healing system
- FWTSD:
-
Flexible wound temperature sensing device
- UV:
-
Ultraviolet
- Si-NMs:
-
Silicon nanomembranes
- BES:
-
Bioabsorbable electronic stent
- HIFU:
-
High-intensity focused ultrasound
- AgNFs:
-
Silver nanofibers
- AgNW's:
-
Zilveren nanodraden
- TLC:
-
Thermochromic liquid crystal
- RF:
-
Radiofrequentie
Nanomaterialen
- Maxim's zorgsensoren voor ultrakleine wearables
- Meelfabriek State-of-the-art gevarenmonitoring voor state-of-the-art korenmolen
- Gecombineerde stroom- en temperatuurfilterbewakingssensoren optimaliseren de processen in de zuivelindustrie
- DIY:temperatuurbewaking en -regeling voor HomeBrew
- Gouden nanodeeltjes voor chemosensoren
- Nanodiamanten voor magnetische sensoren
- Demonstratie van een flexibele op grafeen gebaseerde biosensor voor gevoelige en snelle detectie van eierstokkankercellen
- Draagbare gassensor voor gezondheids- en milieubewaking
- Ultra-high performance flexibele ultraviolette sensoren voor gebruik in wearables
- V&A:Afdrukbare flexibele batterijen
- Ultradunne en zeer gevoelige reksensoren